The Nuclear Conundrum 2023
Alan Journet Ph.D.
Co-facilitator, Southern Oregon Climate Action Now January 2023
It may facilitate understanding of the three main arguments that underlie promotion of the nuclear option to review the basics of atomic structure and nuclear power plant operation, so I begin with a little background. Readers wishing to by−pass this brief discussion of atomic structure, the nuclear reaction, health effects of radiation, and nuclear reactors, can ‘cut to the chase’ by skipping to The Case for Nuclear Power (p. 21).
Background
Atomic Structure
Many readers may already understand that all matter is composed of atoms and that atoms are composed of a nucleus itself comprising one or more of each of (positively charged) protons and (neutrally charged) neutrons, surrounded by a cloud of (negatively charged) electrons. A neat discussion of this is available as a three−and−a−half−minute Nova video (Kestin, undated). Elements in the Universe (carbon, oxygen, nitrogen, gold, nickel, etc., See Table 1) are different and exhibit different properties based on the number of protons present in the nucleus of the atom, the number of neutrons in the nucleus and the number of electrons surrounding that nucleus. An atom is neutral (in charge) if the number of negatively charged electrons outside the nucleus is equal to the number of positively charged protons inside. The atomic number of an atom is the number of protons while the atomic mass is the number of protons plus neutrons. Based on their atomic number, the 118 known elements are arrayed in ascending value in the Periodic Table of the elements (Table 1, modified here from Sharp and Bryner, 2022). The array is determined also by the arrangement of the electrons – a subject more complex to explain than the space available here allows.The elements depicted In Table 1 are:
Row 1 H – Hydrogen; He – Helium
Row 2 Li – Lithium; Be – Beryllium; B – Boron; C – Carbon; N – Nitrogen; O – Oxygen; F –
Fluorine ; N – Neon
Row 3 Na – Sodium ; Mg – Magnesium; Al – Aluminum; Si – Silicon; P – Phosphorus; S – Sulfur; Cl – Chlorine; Ar – Argon.
Row 4 K – Potassium; Ca – Calcium; Sc – Scandium; Ti – Titanium; V – Vanadium; Cr – Chromium; Mn – Manganese; Fe – Iron; Co – Cobalt; Ni – Nickel; Cu – Copper; Zn – Zinc; Ga − Gallium; Ge – Germanium; As – Arsenic; Se – Selenium; Br – Bromine; Kr – Krypton.
Row 5 Rb – Rubidium; Sr – Strontium; Y – Yttrium; Zr – Zirconium; Nb – Niobium; Mo – Molybdenum; Tc – Technetium; Ru – Ruthenium; Rh – Rhodium; Pd – Palladium; Ag – Silver; CD – Cadmium; In – Indium; Sn – Tin; Sb – Antimony; Te – Tellurium; I – Iodine; Xe – Xenon.
Row 6 Cs – Cesium; Ba – Barium; * Hf – Hafnium; Ta – Tantalum; W – Tungsten; Re – Rhenium; Os – Osmium; Ir – Iridium; Pt – Platinum; Au – Gold; Hg – Mercury; Tl – Thallium; Pb – Lead; Bi – Bismuth; Po – Polonium; At – Astatine; Rn – Radon.
Row 7 Fr – Francium; Ra – Radium; **; Rf – Rutherfordium; Db – Dubnium; Sg – Seaborgium; Bh – Bohrium; Hs – Hassium; Mt – Meitnerium; Ds – Darmstadtium; Rg – Roentgenium; Cn – Copernicium; Nh – Nihonium; Fl – Flerovium; Mc – Moscovium; Lv – Livermorium; Ts – Tenessine; Og – Oganesson.
Row 8 (* − insert at Row 6 asterisk above) La – Lanthanum; Ce – Cerium; Pr – Praseodymium; Nd – Neodymium; Pm – Promethium; Sm – Samarium; Eu – Europium; Gd – Gadolinium; Tb – Terbium; Dy – Dysprosium; Ho – Holmium; Er – Erbium; Tm – Thulium; Yb – Ytterbium; Lu – Lutetium.
Row 9 (**insert at Row 7 asterisks above) Ac – Actinium; Th – Thorium; Pa = Protactinium; U – Uranium; Np – Neptunium; Pu – Plutonium; Am −Americium; Cm – Curium; Bk – Berkelium; Cf – Californium; Es – Einsteinium; Fm – Fermium; Md – Mendelevium; No – Nobelium; Lr – Lawrencium.
Isotopes of an element are atoms with the same number of protons (i.e., same atomic number) but a different number of neutrons, thus a different atomic mass.
The following discussion is modified largely from Fuge (2021). Most atomic isotopes are stable, meaning they do not emit nuclear particles. Some isotopes, however, are unstable, meaning they emit nuclear particles through a process known as ionizing radiation or radioactivity. An unstable atom continues to emit particles (or decay) until it reaches a stable configuration. The length of time taken to transform through loss of these particles into another species of atom (with a different number of protons and/or neutrons) is measured in terms of the half−life of the process. This refers to the length of time taken for half of the nuclei to decay to another isotope species (either the same or a different element depending on the number of protons retained). This half−life reflects both the rate of decay and the intensity of emitted radiation; these both halving. Note that the product of this decay may also be unstable and radioactive, and thus itself decay further, emitting more radiation in the process. Decay continues, and radiation Is emitted, until a stable configuration is achieved.
An example from Britannica (undated) illustrates the process:
“The radioactive isotope cobalt−60 [27 protons; 33 neutrons], which is used for radiotherapy, has, for example, a half−life of 5.26 years. Thus, after that interval, a sample originally containing 8 grams of cobalt−60 would contain only 4 grams of cobalt−60 and would also emit only half as much radiation. After another interval of 5.26 years, the sample would contain only 2 grams of cobalt−60. Neither the volume nor the mass of the original sample visibly decreases, however, the unstable cobalt−60 nuclei decay into stable nickel−60 nuclei [28 protons; 32 neutrons; during decay a neutron is changed to a proton], which remain with the still−undecayed cobalt.
Several kinds of radiation emissions can occur during the decay process:
Alpha (α) radiation occurs when an atom loses two protons and two neutrons (interestingly, this alpha particle is equivalent to a Helium atom – see Table 1). Alpha particles cannot penetrate skin but are dangerous if ingested when they can cause cell damage.
Beta (β) radiation involves the emission of a high energy, high speed, negatively charged electron or a positron (a positively charged particle the size and mass of an electron). Because of its small mass, it can travel further in the air and can also penetrate a few centimeters into the skin and thus cause health problems − though these are more severe if the particles are ingested. Beta−decay normally occurs in nuclei that have too many neutrons to achieve stability.
Gamma (γ) radiation usually occurs with alpha or beta radiation but does not consist of particles. Rather, the radiation comprises photons of energy that have zero mass or charge and can therefore travel further. However, they can be stopped by high atomic mass materials such as lead or depleted uranium – hence the lead protective shields when we submit to radiation analysis.
X Rays are similar to gamma radiation but originate from the electron cloud. They are usually longer wavelength, with lower energy than gamma rays.
Neutron radiation occurs when a neutron is emitted from the nucleus, usually because of spontaneous or induced fission of the nucleus (see: the basic nuclear chain reaction under Nuclear Power Generation discussed below).
For additional discussion see Mirion (2015).
Nuclear Power Generation
The essence of the nuclear electricity generation process is a nuclear chain reaction (Figure 1) where the result of fission in one atom, induced by bombardment by a neutron, releases energy and neutrons that collide with other atoms and, in turn, cause them to undergo fission releasing further energy and so on. The basic atom involved in nuclear chain reaction process is a fissile (splitable) isotope of Uranium (U235). Ross (2022) offers a discussion of uranium and its history and role in nuclear reactors and nuclear weapons. When a free neutron collides with an atom of U235(with a nucleus of 143 neutrons and 92 protons, hence the atomic mass of 235), it splits the nucleus and releases energy and neutrons. The most common fission products are atoms with mass numbers around 90 and 137 (NIH 2012) such as Strontium 90 and Cesium 137. The emitted neutrons then collide with other U235 atoms causing further nuclear fissions and so on…., hence the designation ‘chain reaction.’ The main products of the fission reaction (Figure 1) are listed in Table 2 accompanied by their half−lives and decay products. The nuclear chain reaction emits energy as heat. In a nutshell, this heat is then used to boil water, generate steam, and subsequently drive a turbine that generates electricity.
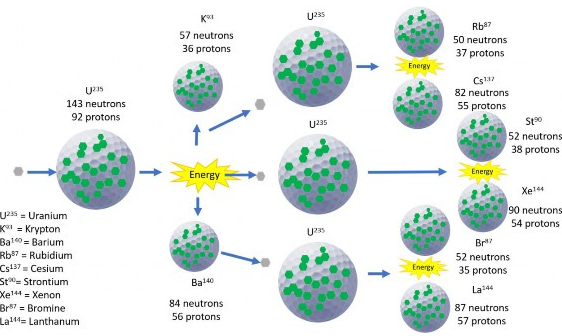
Naturally occurring Uranium is rare, at 4 parts per million, and contains only about 0.7% the critical radioactive U235, the rest being non−fissile U238 (meaning it cannot sustain a chain reaction). As a result, the fuel is enriched by gasification or by means of a centrifuge to achieve 3% to 5% U235. After enrichment, and subsequent use in the reactor, the fuel becomes depleted Uranium since the density of U235 is reduced. This is far less radioactive than the initial fuel and only dangerous if inhaled or ingested. Uranium isotopes have variable half−lives: U238 − 4 billion years; U235 >700 million years; U234 about 25 thousand years.
Depleted Uranium (Depleted UF6 undated) means that the substance emits very low−level radiation but does so for many years (see below). Additionally, this product is extremely tough physically and may be used as tank armor and as casing for armor−piercing shells.
Among the radioactive by−products of fission (Table 2), some are short−lived isotopes while others are very long−lived (Radioactivity, undated−a). Short−lived products vanish in under 5 years; medium−lived products last between 5 and 100 years (Radioactivity, undated−a), while (confusingly) long−lived products are defined as lasting longer than 30 years (Radioactivity, undated – b). This source also notes, however, that rapid decay rates, while leading to the conversion of the isotope to another species quickly, also emit more intense radiation than long−lived isotopes. While emitting for a much longer time, long half−life isotopes emit at a much lower intensity and are thereby less dangerous. A consequence of the rapid decay of the short and medium−lived isotopes is that after 500 years the radioactivity in spent fuel has decreased to a few hundredths or thousandths of the original hazard.
According to Soult (2020), the initial products of the fission of Uranium are: Krypton Kr92 and Barium Ba141; additional fission products are: Cerium Ce144, Praseodymium Pr144, Rubidium The key members of the long−lived group (Table 2) are Strontium−90, Cesium−137, Plutonium−239, Technetium−99, Tin−126, Selenium−79, Zirconium−93, Cesium−135, Palladium−107, and Iodine−129. This table also displays the radiation form and products. The result of their presence is that the radioactivity, though low intensity, reaches (decreases to) a plateau which will last hundreds of thousands of years.
Recall that the enriched Uranium fuel comprises 3 – 5% U235; the rest is U238. When the neutrons produced from fission of the former collide with the U238 this atom is converted to Plutonium (Table 3) which exists as several isotopes (with percentage, half−lives, and decay products as indicated).
The decay of radioactive elements can produce
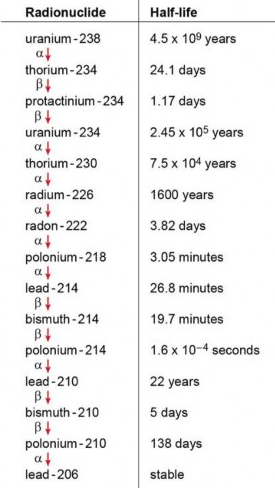
a chain of decay products, each with its own half−life to the next isotope in the chain. For example, the decay chain for Uranium 238, with emissions, is depicted in Table 4. Throughout the sequence, until the final stable isotope is achieved, potentially hazardous radioactive emissions occur.
During normal operations, nuclear power generators release small amounts of low−level radioactive isotopes (EPA 2022a). Additionally, Fast Company (2022) reports that: “the majority of reactors have leaked tritium, a radioactive form of hydrogen that can contaminate drinking water and, at high enough concentrations, cause cancer and genetic defects.”
Controlling the Nuclear Reaction and Meltdown
The chain reaction is regulated by control rods of boron, cadmium, hafnium, or other elements that absorb the neutrons and thus slow the rate of their collisions with uranium or plutonium. If the control rods are removed, the rate of neutron collisions increases, the fission rate increases and energy production and release increase (Libretexts 2021). In addition to the control rods regulating the reaction, the fission reaction is cooled by water pumped around the reactor core (Matson 2011). Optimally, the heated coolant water is cooled and recycled back through the system. Sub−optimally, it is discharged into surrounding waters increasing their temperature and compromising aquatic wildlife species. Matson (2011) continues by explaining that without a steady supply of coolant, the reactor core may continuously emit heat and boil off the coolant. Ultimately, the fuel rods will no longer be immersed, thus allowing the fuel to heat and pool at the base of the reactor container. This hot puddle of fuel may then melt through the steel containment vessel and any additional barriers (hence ‘meltdown’) escaping into the outside world to expose the environment to their radioactive isotope decay products.
“After a severe accident such as a core meltdown (a kind of accident U.S. nuclear regulators call “beyond design basis”), a reactor may emit radiation into the environment, impacting all life and land around it…” (NRDC 2022).
Health Effects of Radioactive Isotopes General Effects.
As with most toxins, the key issues with radiation are the strength of the exposure dose and the nature of the exposure (CDC 2021). Radiation can cause damage to the chromosomal DNA in the nucleus (the genetic material controlling cell development and function) either (a) by breaking DNA bonds and thus causing mutations (DNA reorganizations) that may be cancerous (thus, exposure is especially problematic as cells are dividing), or (b) by breaking bonds in the water surrounding the DNA and producing free radicals – unstable oxygen molecules that can damage tissue. Following radiation damage, cells may (i) repair the DNA and resume normal function, (ii) become permanently altered when there is a risk of cancer (uncontrolled cell division leading to tumors and leukemias, or (iii) be killed resulting in organ failure. Short term intense (high dose) radiation is more dangerous than long−term low−level radiation.
In a discussion of the risks of exposure to radionuclides, EPA (2021a) outlines the main variables imposing health risks:
- The energy emitted by the radiation,
- The type of radiation (alpha, beta, gamma x−rays discussed above),
- How often radiation is emitted,
Whether exposure is external (source is outside the body) or internal (source has been ingested or inhaled) with the latter more serious,
If ingested / inhaled, the rate at which the body metabolizes and eliminates the element,
Whether the isotope accumulates in the body and how long it remains.
Because cell division is the most susceptible phase in the cell cycle, the same source (EPA 2021a) identifies children and fetuses as particularly vulnerable. This is largely because their cells are dividing rapidly.
While there are those who argue that no level of radiation is safe, the fact that we are constantly exposed to low levels of naturally occurring radiation both from terrestrial and cosmic sources leads some authorities (International Atomic Energy Agency – IAEA 1998− 2022) to argue that: “…any exposure above the natural background radiation should be kept as low as reasonably achievable, but below the individual dose limits. The individual dose limit for radiation workers averaged over 5 years is 100 mSv), and for members of the general public, is 1 mSv per year.” Sievert (Sv) is the Standard International unit for measuring ionizing radiation dose, measuring the amount of energy absorbed in a human’s body per unit mass, where mSv − milli Sievert or 1,000th of a Sievert. This is argued by the International Commission on Radiation Protection quoted by IAEA (1998−2022). Meanwhile, after indicating some years ago that there is no safe level of radiation (NAS 2005) more recently the National Academy of Sciences (NAS 2022) urged a $100 million study of the effects of low−level radiation exposure. In the earlier study (NAS 2006) the conclusion was that women and children are more susceptible to ionizing radiation than healthy males. Unfortunately, it is the latter who often stand as the reference case. This means that the sensitivity of the most vulnerable individuals is not the reference case.
Desbiolles et al. 2017 reported on the incidence of cancer in the vicinity of French nuclear power plants. They concluded that generally there was no trend except bladder cancer and that was attributed to two sites with confounding chemical pollution.
Nuclear Isotope Hazards:
As the U.S. Energy Information Agency acknowledges (EIA 2021) “A major environmental concern related to nuclear power is the creation of radioactive wastes such as uranium mill tailings, spent (used) reactor fuel, and other radioactive wastes. These materials can remain radioactive and dangerous to human health for thousands of years.”
“[The] residual radioactivity of fission products is due to a handful of long−lived isotopes. Key members of this group are technetium−99, cesium−135, iodine−129, palladium−107 and zirconium−93. Zirconium and palladium are chemically not very mobile, which leaves cesium, iodine and technetium as the most serious potential troublemakers.” (Radioactivity, undated b).
Iodine 129, with a half−life of 15.7 million years (EPA 2022b) is among 37 isotopes of iodine, only one of which (Iodine 127) is not radioactive. Most of this in our environment comes from nuclear testing in the 1950s.
Iodine-131, with a half−life of 8 days, is the most carcinogenic of the iodine isotopes and can cause burns to the eyes and skin. If ingested, it accumulates in the thyroid increasing the risk of cancer or other thyroid disorders (CDC 2018). Cattle eating grass contaminated with I−131 can pass it on to consumers in their milk.
Cesium 135 has a half−life of 2.3 million years while the half−lives of the other 37 isotopes of Cesium, besides the stable form (Cs133), range from a few days to a fraction of a second. Cs 134, with a half−life of two years, and Cs 137, with a half−life of 30 years are the hazardous isotopes (Chemeurope 1997−2022a,b) which in compounds can bioconcentrate in the body (Science Direct 2022).
Technetium-99 occurs naturally in the Earth’s crusts in very small quantities and besides being produced as a byproduct of nuclear reactions and thus present in nuclear waste, also occurs as a byproduct of nuclear weapons explosions; its half−life is 211,00 years. If ingested, this form of Technetium concentrates in the thyroid and gastrointestinal tract increasing the risk of cancer. Meanwhile, Technetium 99m is a short−lived variant used in medicine with a half− life of 6 hours (EPA 2021b).
Palladium 107 with a half−life of 6.5 million years is the second longest−lived of the 7 long− lived nuclear fission radiation products but is the least radioactive (Chemeurope 1997−2022c). It undergoes pure beta decay to silver.
Zirconium 93 has a half−life of 1.53 million years decaying via low energy beta radiation to radioactive Niobium 93, which in turn has a half−life of 14 years and decays to ordinary Niobium 93 while emitting gamma radiation (Chemeurope 1997−2022d).
Plutonium 239, formed when an U238 atom captures a neutron from the fission process, has a half−life of 24,000 years. Approximately 1.15% of the spent fuel from nuclear generation is
Plutonium while over 1/3rd of the energy produced in a nuclear reactor is derived from Plutonium fission. Weapons grade Plutonium is a by−product of normal reactor operation after three years. Meanwhile, weapons grade Plutonium can be recovered from uranium fuel after 2 −3 months of use in a reactor (World Nuclear Association 2021). This suggests that promoting nuclear energy generation is, indeed, a likely contributor at least to the potential for nuclear weapons proliferation since it provides the fuel. The health risks from Plutonium result from its ingestion, entry via a wound, or inhalation, with the last exposure offering the greatest threat.
According to Jacoby (2020) uranium oxide pellets spend about 5 years in a nuclear reactor by which time their effectiveness declines and they are replaced with fresh fuel. The spent fuel is about 95% uranium, is thermally hot, and also contains a mixture of radioactive Plutonium (Pu244, Pu242, Pu239 with half−lives respectively of 82 million, 380, and 24 thousand years) and other fission products and actinides (a series of 15 radioactive metallic elements with atomic numbers from 89 – 103).
Selenium 79 is not one of the 7 long−lived fission products but is problematic because it is the only one of the nine radioactive selenium isotopes resulting from nuclear fission to be of concern. This concern is based on its half−life of 65,000 years during which it emits beta particles that can be hazardous if ingested (hpschapters 2001).
Types of Nuclear Reactors:
Essentially, the operating principle of the nuclear power plant is no different than that of coal− or gas−powered electrical generation facilities (NRDC 2022) where water is heated to produce steam that drives turbines that rotate to generate electricity.
Lyman (2021) pointed out that the basic nuclear technology employed across the globe is the Light Water Reactor (LWR) which uses conventional water as the basic agent for cooling the core (the container where the nuclear chain reaction occurs). However, about 10% of reactors, he noted, replace light water with heavy water (in which the Hydrogen is replaced by Deuterium having the same atomic number as H, i.e., a single proton, but with a neutron added, thus doubling the atomic mass). Lyman (2021) also indicated that there have been efforts to promote so−called Advanced Reactors that are Non−Light Water Reactors (NLWRs). These replace the water with substances such as liquid sodium, helium gas, or even molten salts. Boiling Water and Pressurized Water reactors (discussed briefly below) are the prevailing types of LWR.
Boiling-water Reactors (Figure 2)
“In a boiling−water reactor, the reactor core heats water, which turns directly into steam in the reactor vessel. The steam is used to power a turbine generator” (EIA 2022a). This, in turn, generates electricity.
Pressurized-water Reactors (Figure 3) This reactor is described as follows (EIA 2022a): “In these generators the chain reaction described above in the reactor core heats water and keeps it under pressure to prevent the water from turning into steam. This hot radioactive water flows through tubes in a steam generator.” This source continues: “A steam generator is a giant cylinder filled with nonradioactive water (or clean water). Inside the giant water−
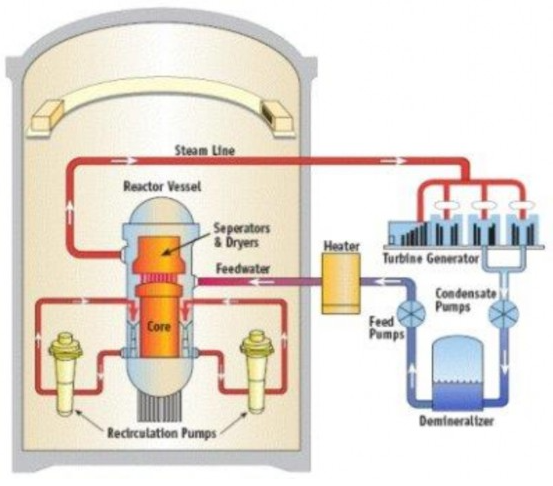
filled cylinder are thousands of tubes filled with the hot radioactive water from the reactor core that eventually bring the adjacent clean water to a boil and turn it into steam.” This comprises the nuclear heat exchange system. The radioactive water flows back to the reactor core to be reheated, and once reheated, returns to the steam generator” (EIA 2022a):
As of July 1, 2022, there were 92 nuclear reactors operating in the U.S. of which 61 were pressurized−water reactors (EIA 2022a). The NRDC (2022) provided a map displaying the locations of these reactors (Figure 4).
The basic nuclear power plant license issued by the Nuclear Regulatory Commission (NRC) is for 40 years, though operators can apply for an extension for another 20 years with no limit to the number of such re−applications (NRDC 2022). This NRDC commentary also points out that the average age of currently operating reactors is 40 years, while some plants are applying for an 80−year license and the NRC is discussing the possibility of 100−year permits.
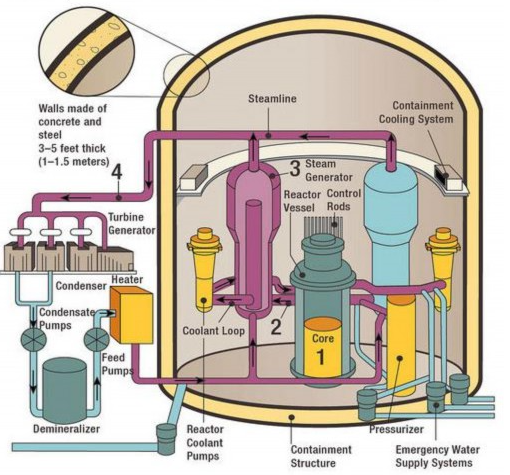
Small modular reactors (SMRs)
According to the U.S. Energy Information Agency (EIA 2022a), these are about a third of the size of currently operational nuclear power plants while those currently under construction are simple and compact in design and can be assembled in a factory and transported to the site. It is suggested that these features may allow more rapid nuclear power plant construction. Whereas large conventional nuclear reactors produce 700
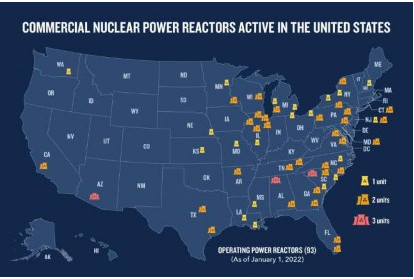
or more Megawatts (MW) of energy, SMRs operate at 300 MW, while the even smaller version Microreactors operate at up to 10 MW (Liou 2021). The same source suggests that SMRs require less fuel and also more infrequent refueling than conventional reactors. However, as of 2021, the International Atomic Energy Agency (Liou 2021) states “their economic competitiveness is still to be proven in practice …”
According to the IAEA’s Liou (2021), SMRs are small reactors with a capacity to generate up to 300 MW per unit (about a third the capacity of traditional nuclear power plants) and produce low carbon electricity. The modular aspect refers to the fact that they are prefabricated off− site and then transported as a unit to the site. Because of their small footprint (area requirement), they can be sited where large conventional nuclear reactors are not possible.
Because of their reduced cost in construction, they can be installed incrementally as energy (electricity) demand increases.
Liou (2021) also pointed out that: “The IAEA expects to publish a Safety Report on the applicability of IAEA safety standards to SMR technologies in 2022.” According to Donavan and Vives (2022) as of April 2022, the IAEA had completed its review of safety standards that would be applicable internationally and was expected to publish a report later in 2022. Liou (2022) then reported that IAEA’s Nuclear Harmonization and Standardization Initiative, charged with developing such standards, with a focus on SMRs first met in June. Since SMR development is occurring in many nations, the discussions involved 133 participants from 33 nations. It is not clear when the necessary codes and standards will appear.
Nakhle C. (2022) indicates that not only does it take on average eight years to build a nuclear power plant, more importantly the time between the decision and the commissioning can vary between 10 to 19 years. On the positive side, Nakhle (2022) argues, SMRs are not suitable for producing weapons−grade materials since uranium enrichment tends to be limited to 20 percent or less, so it is easier for them to comply with nonproliferation regulations.
Furthermore, SMRs have reduced fuel requirements. She reports that the International Atomic Energy Agency (IAEA) stated that power plants based on SMRs may require refueling every three to seven years, compared to every one to two years for conventional plants. Some SMRs are designed to operate for up to 30 years without refueling. The positive implication is that less frequent refueling decreases the risks inherent in transporting radioactive matter.
However, Nakhle (2022) acknowledged that at this stage, SMRs remain mostly a concept and that their economic competitiveness and general viability remain to be tested. She suggests that it is currently difficult to find reliable data demonstrating the commercial potential of this technology. As a result, it’s difficult to offer a forecast.
An important measure of the merit of a technology is the levelized cost of energy (LCOE) of various sources of electricity. This metric incorporates the lifetime, capital cost, operations and maintenance expenses, fuel expenditures and energy production of a technology. Nakhle (2022) reports that one study found that SMRs are in fact the costliest option.
Farmer (2022) pointed out that despite their potential for use where conventional nuclear reactors are not possible, most current plans are to construct very small capacity SMRs on site alongside current reactors. Farmer (2022) also noted that Oregon’s Nuscale SMR company plans to develop SMRs in Poland and build a plant in Idaho starting operations in 2029.
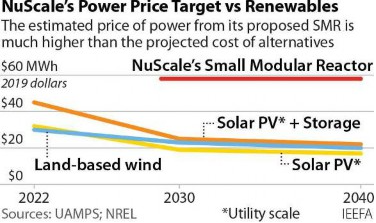
However, Nuscale has attracted critique from the Institute for Energy Economics and Financial Analysis think tank. The institute (IEEFA 2022) reported that the Nuscale design was “too late and too expensive, too risky and too uncertain” compared to modern solar and wind renewables.
Nuscale claims its SMR have a generating cost of $58/MWh, through to 2040 with a construction cost of $3,000/kW. Meanwhile, the IEEFA (2022) cost analysis (Figure 5) reports that the comparable cost for solar and wind energy will be much lower by then.
Additionally, in reviewing the waste production from SMRs, Krall et al. (2022) concluded that in comparison to existing PWRs (Pressurized Water Reactors), SMRs will increase the volume and complexity of LILW (Low and Intermediate Level Waste) and Spent Nuclear Fuel. This increase of volume and chemical complexity will be an additional burden on waste storage, packaging, and geologic disposal.
The discrepancy regarding nuclear proliferation risk may be explained as Virgili (2020) indicates by the fact that some SMRs operate on < 20% enriched uranium, while some use > 20% enriched uranium.
They conclude that of three distinct SMR designs they assessed, that relative to a gigawatt− scale PWR reactor, these reactors “will increase the energy−equivalent volumes of Spent Nuclear Fuel., long−lived LILW, and short−lived LILW by factors of up to 5.5, 30, and 35, respectively.”
Small Modular Reactors seem to offer some advantages over the conventional nuclear reactor, but many questions remain. The evidence presented above suggests that there is little likelihood that SMRs will provide a satisfactory climate remedy.
Advanced Non-Light Water Reactors (NLWRs)
In reviewing Sodium−cooled fast reactors (SFRs), High temperature gas−cooled Reactors (HTGRs), and Molten salt−fueled reactors (MSRs) for the Union of Concerned Scientists, Lyman (2021) reported that the answer to the key question of whether these represent an advance over the conventional Light Water Reactors is: “Little evidence supports claims that NLWRs will be significantly safer than today’s LWRs. While some NLWR designs offer some safety advantages, all have novel characteristics that could render them less safe.”
The Thorium Option
Several years ago (whatisnuclear 2007−2022a), the notion of a thorium−based fission process appeared on the scene as a safer and more reliable approach to nuclear generation than the conventional uranium process. However, it turns out that a number of myths were generated during that promotion (whatisnuclear 2007−2022b, Krahn and Worrall 2016) and the thorium option has since lost support.
According to the World Nuclear Association (WNA 2020), Thorium is a slightly radioactive element existing as Thorium−232. It is not usable directly in nuclear reactors but is fertile meaning not itself fissile but convertible into a fissile atom (NRC 2021). Thus, it must first be converted through irradiation to uranium−233, a process that parallels the conversion of Uranium 235 to Plutonium 239 in conventional reactors. The sources of this transformative irradiation are U−233, U−235 or Pu−239.
They (WNA 2020) identify the disadvantages of thorium as including: the fabrication cost to produce the fissile Plutonium; that U−233 is always contaminated with U−232 having a 69−year half−life and decaying to high gamma radiation emitters such as Thallium−208; that Thorium itself is difficult to recycle due to presence of Thallium−228 (an alpha emitter with 2−year half− life). However, some of these concerns, it is argued, can be overcome in a Liquid Fluoride Thorium Reactor.
Although the Thorium cycle is touted as less susceptible to promoting weapons proliferation, WNA (2020) notes that the U−233 is defined by the International Atomic Energy Agency in the same category as High Enriched Uranium (HEU) meaning (Zielinski 2012) it has been enriched to over 20% of the basic U−235. Naturally occurring Uranium−238, meanwhile, contains only about 0.7% U−235, an insufficient proportion to sustain a nuclear reaction much less create a bomb. Nuclear reactors need at least 3 – 4% U−235, while a bomb requires 90% U−235.
Meanwhile, among the advantages (WNA 2020) are that the Thorium nuclear cycle produces less waste than the conventional reactor.
Krzyzaniak & Brown (2019) argue that the claim that thorium reactors would be more economical than traditional uranium reactors because thorium is more abundant, has more energy potential and doesn’t have to be enriched with the comment is false. They point out that the cost of uranium is a small fraction of the overall cost of nuclear energy since nuclear energy economics are controlled largely by the construction cost of the facility and thorium reactors are no cheaper.
Krzyzaniak & Brown (2019) also argue against the claim that thorium reactor waste is easier to deal with than current uranium reactor waste by pointing out that the thorium−uranium waste product has a similar radioactivity after 100 years and a greater radioactivity after 100,000 years. Meanwhile, in terms of the purported lower nuclear weapons proliferation risk, they report that by−products of the thorium cycle can be attractive for developing nuclear weapons.
In addition, NS Energy (2018) reports that while thorium is abundant, can be used in an array of reactor designs, offers reduced weapons proliferation opportunities compared to conventional nuclear generators, results in reduce hazardous waste production, and is safer to extract, it involves higher start−up costs, requires high temperatures to produce the thorium oxide than is required for producing uranium oxide, and can result in substantial emissions of gamma radiation from the U−232 present in irradiated thorium.
According to Pistilli (2022), since Thorium has been considered an excellent nuclear energy alternative for decades, it’s hard to believe the safety and efficiency benefits have not led to more popular use of thorium reactors. She acknowledges, however, a major reason is that thorium−based reactors are still not economically viable for the most part while Uranium has benefited from decades of research, development and infrastructure thanks to its dual applications in weapons and energy during the Cold War. This research has allowed countries to establish protocols, infrastructure and knowledge bases that make uranium−based energy an easier option. She then concludes that at least for now, thorium reactors are unlikely to gain the upper hand over uranium oxide reactors. It’s possible that thorium reactors could become more dominant in the future, but a lot of work will have to be done to get to that point.
In a discussion of the capacity for thorium to compete with uranium Dumé (2022) states: “Th− 232 is of interest for nuclear power generation because it can easily absorb neutrons and transforms into Th−233. This new isotope emits an electron and an antineutrino within minutes to become protactinium−233 (Pa−233). This isotope, in turn, transforms into U‑233, which is an excellent fissile material. Indeed, the fission of a U‑233 nucleus releases about the same amount of energy (200 MeV) as that of U‑235.” However, she adds: “The U‑233 produced at the end of the cycle is also difficult to handle, as it contains traces of U‑232, which actively emits gamma radiation. While some researchers support the use of thorium as a fuel because its waste is more difficult to turn into atomic weapons than uranium, others argue that risks remain.”
In terms of the capacity to generate weapons−grade material from the thorium reaction, Nuclear Matters (2020) argues that it is a possible pathway but “This process is rarely used because thorium (Th) as a nuclear fuel is less efficient than either natural uranium in a heavy− water reactor or low−enriched uranium in a light−water reactor.” It is also pointed out that “… the uranium−233 produced is less efficient as a fissile material than plutonium…”
The evidence suggests that the benefits of the Thorium cycle have been exaggerated and that this technology offers little advantage over the conventional uranium reaction. As a result of the negatives, the thorium option has more recently lost support.
The cost and timeline for nuclear deployment
Caroline Reiser, a staff attorney with Natural Resources Defense Council’s nuclear team, points out (quoted in NRDC 2022) that nuclear power plants “cannot compete economically with other low−carbon energy sources, like solar and wind, or with investments in energy efficiency.” Reiser continues: “while…advocates argue that nuclear power is important to decarbonizing the economy, it simply isn’t a solution to the climate crisis, especially in the time frame that we need to act.”
Jacobson (2019) underlined this point with the estimation: “New nuclear power plants cost 2.3 to 7.4 times those of onshore wind or utility solar PV per kWh, take 5 to 17 years longer between planning and operation, and produce 9 to 37 times the emissions per kWh as wind.” A 2022 report by the U.S. Energy Information Agency (EIA 2022b) identified the cost of nuclear electricity at some 3 – 4 times that of solar photovoltaic or wind−generated electricity. Interestingly, as an aside, ultra−supercritical coal−fired power plants with 90% carbon capture and storage (CCS – a technology promoted by coal companies as a way to maintain coal in the climate conscious energy mix. See, for example, GE undated appear as expensive as nuclear power.
Nuclear Power Plant Safety Nuclear Waste
As the U.S. Energy Information Agency acknowledges (EIA 2021): “A major environmental concern related to nuclear power is the creation of radioactive wastes such as uranium mill tailings, spent (used) reactor fuel, and other radioactive wastes. These materials can remain radioactive and dangerous to human health for thousands of years.” This discussion continues: “The radioactivity of these wastes can range from a little higher than natural background levels, such as for uranium mill tailings, to the much higher radioactivity of used (spent) reactor fuel and parts of nuclear reactors…..“ Nuclear waste is categorized as low−level waste and high−level waste.
Bemnet Alemayehu, a staff scientist with NRDC’s Climate & Clean Energy Program, quoted by NRDC (2022) stated: “Leading science holds to the linear no−threshold (LNT) model for radiation protection, which assumes that even very small doses of radiation can still increase the risk for cancer….”
By volume, most of the waste resulting from reactor operation is low−level waste (EIA 2021). Low−level waste comprises uranium mill tailings, and the tools, protective clothing, wiping cloths, and other disposable items that become contaminated with small amounts of radioactive dust or particles at nuclear fuel processing facilities and nuclear power plants…” while “High−level radioactive waste consists of irradiated, or spent, nuclear reactor fuel (fuel that is no longer useful for producing electricity)….” (EIA 2021).
In addition, once a nuclear plant has served its purpose for some decades, aged, and its useful life ended, it is closed down. This involves decommissioning during which the structure itself is dismantled comprising: “safely removing from service the reactor and all equipment that has become radioactive and reducing radioactivity to a level that permits other uses of the property (EIA 2021).
The Health Physics Society acknowledge that during normal operation, some (low−level) radioactivity is released (Radiation Answers 2022). However: “The radioactive material is held for a period of time to allow for the radioactivity level to decrease before being treated and/or released in a planned, monitored way.”
According to the Nuclear Information and Resource Service (NIRS undated): “A typical 1000− megawatt pressurized−water reactor (with a cooling tower) takes in 20,000 gallons of river, lake or ocean water per minute for cooling, circulates it through a 50−mile maze of pipes, returns 5,000 gallons per minute to the same body of water, and releases the remainder to the atmosphere as vapor. A 1000−megawatt reactor without a cooling tower takes in even more water−−as much as one−half million gallons per minute. The discharge water is heated and contaminated with radioactive elements in amounts that are not precisely known or knowable but are biologically active.”
Nuclear ‘Accidents’
NRDC (2022) identifies the major historical nuclear incidents:
Since the emergence of nuclear power generation in the 1950s, the worst incident was at Chernobyl in 1986 (then part of the Soviet Union, now, infamously, in Ukraine). Explosions and fire destroyed one of the units following a power surge resulting in radiation reaching as far away as Sweden some 1,000 miles away. Amid a blanket of secrecy that made matters worse, the Soviet Government established an exclusion zone rather than cleaning up the mess.
Thirty−one individuals died and 350,000 were evacuated.
Then, when Russian troops invaded Ukraine in 2022, as the 36th anniversary of the disaster approached, they captured Chernobyl on the first day, set up encampments, and exposed their troops to the radiation still present in that Exclusion Zone (Veranytsia and Veranytsia 2022).
This demonstrates that nuclear waste can never be considered secure. Kinley (2006) reported that: “Doses to the thyroid received in the first few months after the 1986 accident were particularly high in those who were children at the time and drank milk with high levels of radioactive iodine. By 2002, more than 4,000 thyroid cancer cases had been diagnosed in this group, and it is most likely that a large fraction of these thyroid cancers is attributable to radioiodine intake.” Meanwhile, Gale (2021) reporting on longer−term consequences of the Chernobyl incident, stated: “First, there is no question there was an extraordinary increase in thyroid cancers in children and adolescents living in Ukraine, Belarus, and Russia, proximal to the accident.” And “There are about 7,000 excess thyroid cancers, an estimated 100−fold increased incidence, fortunately most not fatal.” Christodouleas et al. (2011) reported the array of radioactive isotopes released as a result of the Chernobyl accident as ranging from molybdenum 99 with a half−life of 67 hours to Plutonium 239 with a half−life of 24,400 years. These authors pointed out that there were “28 deaths related to radiation exposure in the year after the accident.”
There is considerable controversy about the number of deaths attributed to Chernobyl (Gray 2019) with an initial internationally recognized death toll of just 31 and UN estimates of only 50 deaths. However, in 2005, the U.N. predicted a further 4,000 might eventually die as a result of the radiation exposure (Blakemore 2019).
The second worst global event followed a tsunami with 30−foot waves disabling the nuclear facility at the Fukushima Daiichi Nuclear Power Plant in Japan. Although the radiation released resulted in no immediate deaths, over 100,000 residents were evacuated, and the entire nation experienced radioactive fall−out. The economic cost of this disaster is likely to exceed $200 billion. Christodouleas et al. (2011) noted that this incident resulted in the release of radioactive water into the ocean but argued that it diffuses rapidly with distance and decays over time.
Possibly the best−known incident in the United States remains the 1979 Three Mile Island partial meltdown which caused no immediate deaths but resulted in hundreds of thousands of individuals voluntarily evacuating. Clean−up took more than a decade and cost $1 billion in 1993 dollars (over double that in today’s dollars). Evidence regarding short−term increase in cancer risk was inconclusive (Hatch et al. 1990). Of course, immediate deaths are not the only measure of health risk since the latency period between exposure and disease may be several years. Wing et al. (1997) reviewed earlier data and concluded that cancer risk had, indeed, increased downwind of the plant. Meanwhile, in a recent post (TMIA 2019) Wing was reported as stating: “Many earlier researchers, as well as government and industry officials, accept as fact that only small amounts of radiation were released into the atmosphere. But it is known that plant radiation monitors went off scale when the accident started. Plumes containing higher radiation could have passed undetected.” In addition, “I think our findings show there ought to be a more serious investigation of what happened after the Three Mile Island accident,” Wing said.
The nuclear power plants themselves are not the only hazard. In 1979, the same years as the Three Mile Island incident, the largest release of radioactivity in the U.S. occurred when a dam collapsed and released 1,000 tons of radioactive mine tailings into the Navajo Nation near Church Rock, New Mexico (Richards 2013) flowing past the homes of 1,700 Navajo residents. “…by the time uranium mining finally petered out in the early 1980s, hundreds of Indian miners had died from lung diseases and cancers that physicians and secret U.S. Public Health studies linked to the miners’ uranium exposure.” This seems a perfect example of how frontline vulnerable Americans are ignored in debates over safety and health issues.
Events during the Russian invasion of Ukraine at the largest nuclear power plant in Europe (Zaporizhzhia) reveal serious dangers during military conflict (UN News 2022). Regardless of who is responsible for the shelling of the plant, we are seeing that nuclear power plants can be:
- designated as military targets,
- threatened with attack,
- or used as shields from which to launch offensive missile strikes.
The abuse of nuclear facilities by terrorists and in times of military conflict cannot be ignored, an especially critical problem if nuclear reactors are scattered around the planet in areas of the world exhibiting civil unrest and political instability. Promoting renewable energy generation facilities would be far safer than promoting nuclear generation in such locations.
Finally, the fact that conventional nuclear power plant operation can produce fuel for nuclear weapons, as discussed below, adds substantially to the risk of this technology.
Nuclear Weapons Proliferation
The product of conventional Heavy Water and Light Water nuclear reactors is Plutonium an atom that can be fabricated into the necessary fissile component for nuclear weapons.
Schellenberger (2018) pointed out that “Of the 26 nations around the world that are building or are committed to build nuclear power plants, 23 have a weapon, had a weapon, or have shown interest in acquiring a weapon…” There is an obvious risk in promoting nuclear reactors as global sources of energy in that this inevitably places this technology and nuclear proliferation in the hands of potentially unstable nations across the globe, vastly increasing the risk of nuclear conflict
The Price Anderson Act
This Act, initially passed by Congress in 1957 and since renewed, provides the nuclear industry with a substantial subsidy that tilts the energy economic playing field in its favor.
As described by Holt (2018) the Price Anderson Nuclear Industries Indemnity Act authorizes the Nuclear Regulatory Commission to limit the liability of nuclear licensees from radiation damage to the public. This authority has been extended by Congress four times; it currently remains in effect until 2025. The Act requires nuclear generator owners (1) to carry insurance liability up to the current commercially available maximum, ($450 million as of January 1, 2017), (2) for owners of 100−megawatt−and−above power reactors to contribute to an industry−wide fund which covers damages above $450 million through a contribution by each nuclear reactor owner of up to $121.3 million. As a result of the number of reactors liable for this payment, the total in this fund caps at $12.4 billion but it is variable depending on the number of liable reactors. Damages above this amount would require Congressional action to be funded, but there is no source for any such funds, so these would come from general revenue (i.e., the U.S. taxpayer).
It’s worth being reminded that restoration of damage from the Fukushima incident is anticipated to cost over $200 billion. Thus, if a Fukushima−like incident were to happen in the U.S. taxpayers would be responsible for over $190 billion.
Holt (2018) also notes: “The Price−Anderson Act’s limits on liability were crucial in establishing the commercial nuclear power industry in the 1950s. The nuclear power industry still considers them to be a prerequisite for any future U.S. reactor construction.” We live in a society where individuals and businesses are, in many cases, required to carry insurance to cover damages should they be responsible for an accident. Surely, by the same token, an industry that claims to be safe should be required to negotiate insurance from private insurers. If that industry cannot persuade insurers to provide coverage, or afford the premiums levied, the message about safety of operations at nuclear reactors should be clear.
Polonsky & Eskelsen (2021) report that the Nuclear Regulatory Commission sought no changes in the liability plan when the Price Anderson Act was considered by Congress that year.
In 2021, the Department of Energy initiated a public comment period preparatory to a review of the act (Fork and Fowler 2021). These authors noted that the Price−Anderson Act is critical to nuclear suppliers’ ability to manage their risk. In its report on recommendations regarding re−authorization, the Nuclear Regulatory Commission authors (USNRC 2021a) pointed out that the maximum coverage available per incident from this Act is $13.4 billion. Since this total is based on the per reactor allocation to the fund, if the number of reactors decreases from the total (94 as of October 2020) the total funds available would decrease since each reactor contributes $137.609 million to the fund. Despite the cost of the Fukushima incident estimation of over $200 billion, this report relies only on the U.S. history of claims and the Three Mile Island example to conclude that the total available from the fund is sufficient to meet needs. This conclusion is offered despite the recognition that with nuclear plant retirements, this total number of reactors will likely drop, as will the amount in the fund. The key point not mentioned is that any accident cost beyond the fund that stands at a little over 1/10th the Fukushima cost will be covered by the U.S. taxpayer. In reviewing the NRC report, Lewis (2021) summarized it as follows: “The NRC … does not recommend repealing or modifying any specific provisions, though it does make a few minor recommendations relating to the treatment of nonprofit operators and international coverage.” The modifications include recommending a 10−year rather than a 20−year extension based on the anticipated deployment of advanced reactor designs.
It is not clear what Congressional action has occurred in relation to the proposed re− authorization.
The Case for Nuclear Power
Over the years of our awareness of the developing climate crisis, there have been repeated efforts to promote nuclear energy as a solution, or a major contributor to the solution. Some of these efforts have been clearly promoted by the nuclear industry itself apparently trying to regain or increase its share of the energy market. Other efforts have been orchestrated by well−meaning individuals who genuinely see nuclear generation of electricity as having a substantial and beneficial role to play in fighting the climate crisis. Some have even interpreted the evidence to suggest it is essential if we are to succeed because we cannot achieve our global energy needs on renewable sources and storage alone.
Not surprisingly, the nuclear issue is not as clean as many proponents and opponents would have us believe.
Claims that we should promote nuclear energy seem to be based on several premises that deserve evaluation. The three main premises discussed here are as follows:
- Clean renewable energy sources are inadequate to provide our energy needs.
- Nuclear energy generation is free of greenhouse gas emissions.
- Nuclear energy is safe.
To these, we might add three other considerations some of which have been discussed above:
- There are new generation designs that are superior to the historic light water reactor design.
- Failing to support aging nuclear reactors will likely result in their replacement by natural gas generators.
- Economics and length of time to construction and function of nuclear facilities render them of questionable value in combatting the urgent climate crisis.
Evaluating these claims reveals a complex morass of sometimes conflicting evidence. This discussion will try to summarize the evidence as of December 2022.
Clean renewable energy sources are inadequate to provide our energy needs
In a recent report, Jacobson et al. (2022) analyzed the potential for genuine clean renewable energy – defined as energy: “that is both clean (emits zero health and climate affecting air pollutants when produced or consumed) and renewable (has a source that continuously replenishes the supply).” These energy sources mainly comprise Wind, Water and Solar energy with storage but include limited geothermal where available (see, for example, Solutions Project 2022). They do not include “fossil energy, bioenergy, non−hydrogen synthetic fuels, blue hydrogen, carbon capture, direct air capture, or nuclear energy, since each may result in a greater risk of air pollution, climate damage, and/or energy insecurity.” The only form of Hydrogen they considered was Green Hydrogen, that is Hydrogen produced by electrolysis using Wind, Water, or Solar (WWS) energy sources.
The authors noted that as of December 2021, 15 U.S. states, districts or territories had already established a 100% renewable energy goal (California, Connecticut, Hawaii, Maine, Nevada, New Jersey, New Mexico, New York, Oregon, Puerto Rico, Rhode Island, Virginia, Washington D.C., Washington State, and Wisconsin). If the nation were converted to 100% WWS energy by 2050/2051 some 4.7 million more long−term full−time jobs would be created than following a Business−as−Usual scenario involving accelerating fossil fuel use and consequent accelerating greenhouse gas emissions. The land area required for this system would be 0.84% of the current national land surface, a figure which compares favorably with the current 1.3% of U.S. land surface utilized by the fossil fuel industry. Indeed, this represents but 65% of the current land area allocated to energy production.
A report by Bond et al. (2021) for Carbon Tracker concluded “With current technology and in a subset of available locations we can capture at least 6,700 PWh per annum (Picawatt (PWh) = 1015 watts) from solar and wind, which is more than 100 times global energy demand. This opportunity presents itself because the cost of renewable energy has dropped. They also concluded that providing energy from solar alone would occupy only 0.3% of the land surface and that pricing will mean that: “fossil fuels will be pushed out of the electricity sector by the mid−2030s and out of total energy supply by 2050.”
Jacobson’s (2020) book, based on his Stanford University course, offers evidence that the world can be powered 100% on “clean, renewable wind−water−solar (WWS) energy and storage for everything.” He also argued that “The main obstacles appear to be social and political.”
In a study funded by the German Federal Environmental Foundation, Ram et al. (2019) concluded “A global transition to 100% renewable energy across all sectors – power, heat, transport and desalination before 2050 is feasible.” Furthermore, they indicated this is possible with “…e[E]xisting renewable energy potential and technologies, including storage.” They further conclude that a “sustainable energy system is more efficient and cost effective than the existing system, which is based primarily on fossil fuels and nuclear.” In assessing some proposed options, they state their proposed route “…achieves a cost decline without the reliance on high−risk technologies such as nuclear power and carbon capture and [storage] sequestration (CCS). A full energy transition to 100% renewable energy is not only feasible, but also cheaper than the current global energy system.” The obstacle, they suggest, is neither technological feasibility nor economic viability, but political will.
The evidence that wind, water and solar can provide our energy needs has been offered for several years (e.g., Jacobson et al. 2015). Although that paper received some criticism (Clack et al. 2017) the authors responded effectively (Jacobson 2017, Jacobson et al. 2017). Indeed, Jacobson (2017) also pointed out that “most of [the authors of the critique] have a history of advocacy, employment, research or consulting in nuclear power, fossil fuels or carbon capture. Through The Solutions Project (2022), Jacobson and his team have developed road maps for achieving 100% clean renewable energy economies in all 50 states and the nation as a whole as well as most other nations across the globe.
In a review of our energy future, energy and environment economist Mark Cooper (2021) argues that there is no benefit to continuing to subsidize and promote nuclear energy since the ‘nuclear renaissance’ has failed for multiple reasons. Amory Lovins (2021a) from RMI (formerly Rocky Mountain Institute) argued that proponents of nuclear power frame the issue incorrectly by focusing only on carbon and ignoring the issue of cost. Because clean renewables are cheaper, he argues, they displace less carbon per dollar than nuclear energy. Additionally, Lovins points out that most of the carbon emissions reductions in the U.S. to date have resulted from increased energy use efficiency and the increasing role of clean renewable energy with nuclear playing a very minor role. Meanwhile, in a different discussion (Lovins 2021b), he addresses twelve myths that include some promoted by nuclear proponents. Notably, he points out, in response to the criticism that solar and wind electricity are intermittent, that the grid doesn’t result in one generation source providing one user.
Rather there is an array of generators covering a wide array of locations. Although it is certainly true that the sun sets and thus compromises solar generation overnight, as Torchinsky (2022) noted, a recent development in the technology shows promise of solar cells that can generate electricity at night by generating “electricity from the small difference in temperature between the ambient air and the solar cell itself.” Meanwhile, a lull in wind turbine generation in one location can be compensated by generation elsewhere.
Furthermore, the battery storage technology is advancing rapidly and compensating for intermittence involves more options than just batteries.
In addition, there are other techniques available for storing energy: already in use is the water storage system where water is pumped to a high elevation reservoir when energy is abundant, and then runs back down generating energy when the intermittent source is unavailable (e.g., EERE Undated a, EERE Undated b ). An alternative, where local topography is not conducive to the water storage approach, energy can be used to raise a unit of mass (soil or rocks) which then can be lowered to emit the potential energy locked into the elevated mass (e.g., Moore 2021).
In an analysis of forecasts about the energy transition, Way et al. (2021) argued: “Most energy−economy models have historically underestimated deployment rates for renewable energy technologies and overestimated their costs.” They concluded that: “compared to continuing with a fossil−fuel−based system, a rapid green energy transition will likely result in overall net savings of many trillions of dollars − even without accounting for climate damages or co−benefits of climate policy.” Furthermore, they argue that because of the rapid decrease in renewable energy costs and their rapidly increasing deployment an energy future that relies on solar photovoltaics, wind, batteries and hydrogen electrolyzers is preferable because, “In contrast, a slower transition (which involves deployment growth trends that are lower than current rates) is more expensive and a nuclear driven transition is far more expensive.” Again, we see evidence that clean renewable resources are expanding rapidly and offer a more cost− effective approach than promoting nuclear energy.
Premise 1 Inference: There seems abundant evidence, from many independent sources, that this premise for nuclear power, probably the most critical of all, is false. Rather, there is sufficient clean renewable energy to supply our needs. Since Premise 1 is the main premise underlying the argument that we need nuclear energy, its falsification constitutes a substantial blow to the entire argument that nuclear energy is necessary.
Nuclear energy generation is free of greenhouse gas emissions.
When we undertake assessments of the climate impact of our activities, we must do more than examine day−to−day operations. We must examine the full life cycle emissions of that activity. In the case of solar and wind energy, this means examining the emissions that result from the extraction of materials and construction of the solar panels and wind turbines, plus emissions resulting from their transport, installation and maintenance, and finally those resulting from their end−of−life removal and disposal. Fortunately, operation of these renewable generation sources is emissions−free. In the case of nuclear energy, this also means we must include the emissions resulting from the construction, operation, and decommissioning / disposal of the power plant, plus the emissions resulting from the extraction, processing and final disposal of the nuclear fuel. Fossil fuel−powered generation facilities are subject to the same assessment as the nuclear generator. Only when we are armed with these data are we able to make a legitimate comparison.
As an aside, it is worth noting that it’s the failure to undertake full life cycle assessment that allows methane (natural gas) proponents to claim that fuel is ‘the clean fossil fuel.’ In fact, full life cycle assessment reveals that natural gas is as bad or worse than coal in terms of its full life cycle emissions. For more information on this, visit: The Carbon Mistake, The Natural Gas Conundrum, What’s Up With RNG?.
Over the years, several reports have been issued presenting life cycle emissions of various energy sources. These generally report emissions in grams (g) of carbon dioxide equivalent (CO2e) emitted per kilowatt hour (kwh) of electricity generated. Carbon dioxide equivalent is a measure of the warming impact of all gases assessed in terms of their equivalence to that of carbon dioxide – designated as 1. All analyses reveal that fossil fuels result in huge emissions in the many hundreds to thousands of g CO2e/ kwh. When Carbon Capture and Storage technology (CCS) is included, the emissions only drop slightly. This is not surprising, especially when one considers that CCS only addresses combustion emissions so upstream (extraction processing and distribution) emissions are untouched, as are those resulting from willful / unintended emissions from incomplete or inoperative flaring of methane. Given that substantial CO2e emissions from natural gas usage result from methane leakage upstream, or emissions resulting from unintended / willful incomplete or inoperative flaring, CCS can do little to reduce the climate pollution caused by this fuel. A National Energy Technology Laboratory report (Skone et al. 2015), for example, indicated that in the Appalachian Basin, 77% of the CO2e footprint of natural gas comprised methane with the majority of this resulting from distribution, transmission, and well completion. Presumably because the majority of CO2e emissions in the coal cycle result from combustion, imposing 90% carbon capture reduces coal−fired electricity emissions much more than it reduces gas−fired electricity generation emissions. Interestingly, this analysis reports the emissions from nuclear, hydroelectric, wind and solar in the range of 20 – 40 g CO2e/ kwh, while, at 250, geothermal is 6 to 10 times greater. Meanwhile, without CCS, coal is assessed at 1,205 g CO2e/ kwh, Petroleum at 1180 g CO2e/ kwh, and natural gas at 523 g CO2e/ kwh.
Jacobson (2020) also assessed the life cycle emissions of various fuels and concluded that nuclear power emits between 9 and 37 times more greenhouse gases (measured as CO2e) than wind power.
In an early literature review Sovacool (2008) summarized complete life cycle assessments of greenhouse gas emissions measured in g CO2e per kwh electricity generated. With numbers rounded, that report wind (onshore and offshore respectively) at 9 and 10, solar thermal energy at 13 with photovoltaic solar at 32. Various nuclear reactor types averaged out at 66. At that time, the evidence suggests, nuclear was not assessed as equivalent to clean renewable energy sources in terms of emissions. These values compared with biomass (14 – 31) natural gas (443), diesel and oil (998) and coal (960, 1050) revealing how appalling all fossil fuels are by comparison. Additionally, since then, the warming impact of methane has been reevaluated time and again, and each time seems to be revealed as worse than previously thought. It’s worth noting, also, that some of these assessments date from before the fugitive emissions (leakage) of methane in the extraction, processing, and transmission of natural gas were fully assessed and reported. These analyses have consistently demonstrated that natural gas is comparable to other fossil fuels in life cycle greenhouse gas emissions. Alvarez et al. (2018) reported that fugitive methane emissions produced a global warming impact equivalent to the combustion carbon dioxide emissions of the gas – negating the saving gas is often argued to exhibit because when we only consider combustion emissions, we find that emissions per unit of energy generated are lower for methane than for coal and oil. This would likely nearly double the natural gas impact reported above by Sovacool (2008). Indeed, Howarth (2015), a pioneer in the arena of life cycle assessment of the greenhouse gas emissions from natural gas, suggested that a main result of the inclusion of fugitive emissions is to reveal both shale−fracked and conventional natural gas produce a greater number of grams of carbon dioxide equivalent per mega Joule of energy generated than either coal or oil. Natural gas (methane) is not ‘the clean fossil fuel.’
Chapter 7 of the Intergovernmental Panel on Climate Change evaluation of various energy sources (Bruckner et al. 2014) reported lifecycle assessments in a range of 675–1689 g CO2e/kWh electricity for coal. Corresponding ranges for oil and gas were 510–1170 g CO2e/kWh and 290–930 g CO2e/kWh14. They identified the ranges for lifecycle greenhouse gas emissions as 18–180 g CO2e/kWh for Photovoltaic panels, (Kim et al., 2012; Hsu et al., 2012), with 9–63 g CO2e/kWh for Concentrated Solar Power (Burkhardt et al., 2012), and 4– 110 gCO2e/kWh for nuclear power (Warner and Heath, 2012). Wind generation was graphed in the range of solar and nuclear, but the actual value was not reported.
Evans (2017) reported on CO2e emissions in a Carbon Briefs report from a publication by Pehl et al. (2017) using the same units (i.e., g CO2e per kwh electricity generated) as employed by Sovacool (2008) above and others, below. This assessment identified wind at 4, solar at 6 and nuclear at 4 g CO2e per kwh. Meanwhile, coal with Carbon Capture and Storage (109), natural gas with Carbon Capture and Storage (78), Hydro (97) and bioenergy (98) are all over an order of magnitude worse in terms of emissions.
The National Renewable Energy Laboratory (NREL 2021) reported life cycle assessments also measured in terms of g CO2e/kWh, though some were from much earlier studies dating from as far back as 2005 – presumably when no more recent study has been performed. Wind and nuclear tied at 13 g CO2e per kwh with concentrating solar power at 28 and photovoltaic panels at 43. Meanwhile, natural gas, oil, and coal respectively scored 486, 840, and 1001 g CO2e per kwh.
Jacobson (2019) further reported the 100 year life cycle assessment in terms of g CO2e/kWh as follows: rooftop solar 0.8 – 15.8, solar photovoltaic utility 7.85 – 26.9, concentrated solar power 8.43 – 25.2, onshore wind 4.8 – 8.6, offshore wind 6.8 – 14.8, hydroelectric 61−109,
wave 26 – 38, tidal 14−36, nuclear 78 to 178 g, biomass 86 – 1,788, natural gas with CCUS at 230 – 481, and coal with CCUS 282−1,011 CCS where U represents Carbon Capture and Storage with Utilization. Unfortunately, the main utilization in CCUS of the gas is to promote further extraction of fossil fuels and thus generates further greenhouse gas emissions, which rather defeats the purpose.
Jacobson (2020) departed from the pattern of reporting nuclear generation as similar to solar and wind in terms of emissions per unit of energy generated. Rather, that author identified nuclear generation as producing between 9 and 37 times more CO2e and pollution than wind generation. The above data reveal that the range for solar means that nuclear could compare even less favorably.
While substantial differences exist among the studies, presumably based on slightly different methodologies, comparisons within studies reveal that nuclear generation, while consistently much lower than fossil fuels, is never a zero emissions process. It is not entirely clear if all life cycle assessments of the nuclear technology include decommissioning and waste storage, though they should. A study by Koltun et al. (2018) of a so−called fourth generation reactor (gas turbine technology with modular helium reactor GT−MHR) specifically included both decommissioning and waste treatment and revealed g CO2e/kWh of 15, well in line with the data reported above suggesting maybe these components are included.
Premise 2 Inference: While there seems little doubt that nuclear generation is a substantial improvement over coal, oil, and natural gas, at best, it appears to be right in line with the genuinely clean renewable sources of solar and wind. At worst, it simply may not achieve their low emissions so is no improvement over clean renewable sources. Furthermore, investment in nuclear energy would compete with investment in genuinely clean energy sources; every dollar spent on promoting nuclear energy is effectively a dollar subtracted from promoting renewable energy / storage. Meanwhile, as Matthews 2022 point out, the cost per megawatt hour of electricity generated (in 2021 dollars) is as follows: Solar $36.49, Geothermal $29.82, Onshore wind $40.23 while hydro is $64.27, ultra−supercritical coal is $82.61, advanced nuclear is $88.24, and biomass is $90.17. The cost of nuclear power alone renders it non− competitive.
Nuclear energy is safe
There exist two basic concerns regarding health and safety: one deals with the day−to−day operations (including waste production), the other with unpredictable events (whether human−induced or natural).
The Health and Safety Concern
That nuclear fission poses potential health and safety risks is well known. As discussed above (Health Effects of Radioactive Isotopes), the problem with nuclear radiation from unstable isotopes is its proclivity for inducing cancer in exposed organisms. Exposure to the high energy radiation disrupts DNA in the nuclear chromosomes of the cells of exposed individuals, often causing cancerous hard or soft tumors.
As also discussed above, the risk posed by these isotopes depends largely on their half−lives: isotopes with short half−lives tend to emit intense radiation, while those with longer half−lives emit less intense radiation, but obviously do so over a much longer period.
It seems that the normal activity of a nuclear power plant will generally pose little threat to the environment though the heated water discharged from a plant may well disrupt local aquatic species. Problems arise, however, when normal activity is undermined – whether by human error, natural catastrophe (such as earthquakes and tsunamis), or terrorist/military assault.
However, it is worth remembering, as stated by NRDC (2022): “Current radiation protection standards are based on the premise that any exposure to radiation carries some risk, and that that risk increases directly with dose of exposure.” In a significant analysis of the literature, the National Academy of Sciences (NAS 2006) offered: “Epidemiologic studies … show that exposure to low… radiation can lead to the age− and time-dependent development of a wide range of tumor types that, in general, are not distinguishable from those arising in non−irradiated populations”
Richard Clapp, a retired professor from Boston University’s School of Public Health offered in a guest editorial in Environmental Health Perspectives (Clapp 2005) “Given the availability of alternative carbon−free and low−carbon options and the potential to develop more efficient renewable technologies, it seems evident that public health would be better served in the long term by these alternatives than by increasing the number of nuclear power plants in the United States and the rest of the world.”
Responding to an article promoting nuclear power in the energy mix, Larsen (2020) wrote:”…the proponents of nuclear power …are overlooking the significant risks inherent in the technology and the fact that scaling up nuclear power would take too long and is too costly to be an effective climate solution.”
During the Russian invasion of Ukraine, invading forces took over the Ukrainian Chernobyl nuclear disaster site and apparently unwittingly exposed themselves to radioactive hazards. The invading Russian forces then targeted the largest nuclear power plant in Europe, in Zaporizhzhia, with shells and missiles before commandeering it. These events should be enough to alert everyone that nuclear facilities are sitting ducks for ignorant military or terrorist behavior and thereby pose an ongoing threat to citizens within many miles of the facility.
In responding to the claim of operational safety, frankly, it seems that no argument is really necessary except the single word: ‘Zaporizhzhia.’ It has long been suggested that a major threat posed by nuclear power plants is their exposure to terrorist action. Now, with the Russian invasion of Ukraine we have the perfect example of that threat as Putin’s forces attacked the largest nuclear power plant in Europe and with its bombardment risked an outcome potentially equal to Fukushima. Given the number of unsettled regions around the globe, where civil unrest is possibly simmering just below the surface, the expansion of nuclear power, with its capacity to provide fuel to allow nuclear weapons proliferation, seems unwise at best. And if we acknowledge that this energy source is unnecessary, the notion of promoting nuclear power seems downright foolhardy.
It should be acknowledged, however, that maybe the Zaporizhzhia incident, emerging as a result of the Russian invasion of Ukraine is as strong an argument against a large, centralized power generation grid system as it is an argument against the energy resource used in that generation facility. The fact that the Ukrainian power plant is a nuclear facility simply compounds the risk.
Extending Aging Reactor Licenses
The number of nuclear reactors in the U.S. peaked in 1990 at 112 but has since declined as reactors age and competition from cheaper fossil fuels encroaches on profitability (Clemmer et al. 2018). They also point out that most U.S. reactors have 60−year operating licenses.
Lyman (2019) pointed out that as nuclear reactors age, “they require more intensive monitoring and preventive maintenance to operate safely.” Lyman continues with the following comment: “Given that older reactors require more attention from the regulator, not less, it is perplexing that the NRC wants to scale back its inspections of the aging reactor fleet and its responses to safety violations.” From a greenhouse gas emissions perspective, the problem is clear, as indicated by Rivero (2022): a quarter of nuclear capacity in advanced economies is expected to shut down by 2025. The problem is that low fossil fuel prices – exacerbated by the glut of natural gas – make ongoing nuclear operation less cost competitive. Because of the greater greenhouse gas emissions that result from natural gas (methane) electricity generation, the threat that nuclear capacity will be replaced by natural gas generation facilities is a serious problem.
The threat of this transition is exacerbated by lack of nuclear profitability. As Clemmer et al. (2018) pointed out, a third of U.S. nuclear power plants are either unprofitable (16) or scheduled to close (5) a number that means most reactor owners hold unprofitable plants. This lack of profitability would presumably be exacerbated if nuclear entities were required to fund their own insurance completely. They report the conflict between studies suggesting on the one hand that renewable resources and energy efficiency can achieve substantial emissions reductions while current reactors continue operation through their 60 years of licensing and studies, on the other hand, suggesting nuclear power will make a meaningful contribution to reducing emissions while assuming reactors will continue through and beyond their 60 years and the cost of new power plants will decline. However, as they note, predictions regarding nuclear costs have been notoriously underestimated by the industry.
The claim that nuclear power would be “too cheap to meter” (USNRC 2021b) was the first and most notable such overly optimistic claim. This should alert us to be skeptical about any claims of cheap energy offered by the nuclear industry. In an industry that is of questionable profitability where maintaining safety involves considerable expense, there exists considerable risk that safety will be sacrificed on the altar of profitability.
In assessing the future energy needs in this Union of Concerned Scientist (UCS) report, Clemmer at al. (2018) point out that while nuclear power accounted for 53% of low−carbon electricity in 2017, renewable energy was the fastest growing energy source. They also point out the need to replace high−emission sources with low−emission sources. For this reason, the UCS argues that a series of steps should be taken – including, but not limited to – support for maintaining aging nuclear power plants:
- State and Federal policies should support all low−carbon technologies
- Renewable energy and efficiency standards should be adopted
- A robust greenhouse gas emissions pricing system should be imposed
- Financial support is conditioned on consumer protection, safety requirements, and investment in renewable energy efficiency
- A low−carbon emissions standard should be established
- Power plant owners requesting financial assistance should be financially transparent and open their books
- Financial support for non−profitable power plants should be limited
While the UCS endorsement of nuclear generation was greeted enthusiastically by nuclear advocates, in promoting it they often overlook the array of caveats that accompany that endorsement. Especially overlooked are the correlated proposals to limit support for non− profitable nuclear power.
While the problem of aging nuclear plants being replaced by fossil fuel plants is real, the burgeoning availability of renewable energy and efficient battery storage suggest that, if undertaken, support for nuclear power should be employed on a short−term basis only and should not apply to new nuclear plants.
A Note About Fusion
Anyone paying attention to science and energy news in late 2022 will have heard excited commentary about the progress towards nuclear fusion, a process that produces energy by combining light atoms (such as Hydrogen) rather than through nuclear fission of heavy atoms (such as Uranium and Plutonium). The catch, as Stallard (2022) describes the process, is the energy required to drive the process. Thus, the fusion process requires extremely high temperature (of the order experienced on the sun) accompanied by extremely high pressure. On the positive side, however, the energy is produced with a very low radioactive product and no greenhouse gases (so long as the energy used to drive the fusion process is clean).
US DOE (2022) explains the process as follows: an atom of Tritium (an isotope of Hydrogen containing two neutrons and 1 proton) is fused with Deuterium (another isotope of Hydrogen containing 1 neutron and 1 proton). In contrast the dominant and basic Hydrogen atom contains 1 proton and 1 electron but no neutrons (Brittanica 2022); the other isotopes are less common. The US DOE (2022) notes that when the Tritium and Deuterium combine, they produce Helium with two protons and two neutrons meaning one neutron disappears. One neutron is expelled but some mass is lost, and this unit of mass is converted by the process into energy – hence the energy production of nuclear fusion. The reader might recall the Einstein principle that mass and energy are interconvertible (e.g., Fernflores 2019). Different atoms could be used in fusion, but the deuterium – tritium fusion releases most energy and can be conducted at lower temperatures than other fusions.
As Morelle (2022) points out, the experimental success is that the Lawrence Livermore National Laboratory in California has, for the first time, produced more energy than was consumed. To be sure, it was only enough to boil a few kettles, but it was a first step. From a purely scientific perspective, this is notable! The real question, however, is: can this become commercially and globally available within the time needed to address the climate crisis? This means within the next three decades. Unfortunately, turning this experimental procedure to a commercial venture that is readily available will take time. For many years, Morelle (2022) notes, 50 – 60 years has been the answer to the question: “how long?”
Morelle (2022) also notes an additional caution: despite the hype, we should recognize that the excess energy produced did not account for the energy needed to make the lasers work. When this is included, the procedure is no longer positive. While this fusion success is, indeed, a breakthrough, we should again recall the infamous 1954 quote (Terzic 2018) from then Chairman of the Atomic Energy Commission Lewis Strauss that nuclear fission energy would provide energy that is “too cheap to meter.” Yet, nuclear generation still requires huge taxpayer subsidy and even then often does not break even economically. The likelihood seems remote that nuclear fusion can become broadly available on a global scale to put much of a dent in the cause of the climate crisis in the time necessary.
Concluding Remarks and Summary
In a sense, we can already assess what role nuclear energy might play in promoting a low emissions economy since among developed nations there exists a wide range in the degree to which electricity generation is driven by nuclear sources. If nuclear generation were to contribute substantially to lowering a nation’s emissions, this should be reflected in the relationship between nuclear emphasis among nations and the greenhouse gas emissions of those nations. Sovacool (2021) reported on this relationship and concluded that, in fact, greater emissions are associated with those nations that have more nuclear generation than those with less, a result contrary to the expectation if nuclear generation were to reduce emissions. Meanwhile, Sovacool (2021) also points out, nations with a greater emphasis on renewable generation exhibit lower GHG emissions than those utilizing nuclear generation.
Rather than promoting emissions reductions, a nuclear emphasis seems merely to compete with and replace renewables. Maybe promoting nuclear energy psychologically encourages a ‘business as usual’ attitude among users that results in excessive energy utilization and undermines the encouragement of energy use efficiency and conservation. Since we know that there exists no totally benign energy source, actions that promote false solutions and create the impression continued massive energy use is now acceptable are more dangerous than they might initially appear.
Sovacool (2021) adds the caveat that the lead time necessary to bring nuclear power plants online compared to that for renewable generation, indicates that, unlike renewables, nuclear generation is unlikely to be capable of addressing the climate crisis in the time necessary. The same author also notes that the International Energy Agency estimates the cost for installing sufficient nuclear capacity to address the problem globally would be $4 trillion.
This analysis invites an obvious question: if we can provide our energy needs with genuinely clean renewable energy, why invest in, and subsidize, nuclear energy?
The 2014 Intergovernmental Panel on Climate Change AR5 report (Bruckner et al. 2014) states: “Continued use and expansion of nuclear energy worldwide as a response to climate change mitigation require greater efforts to address the safety, economics, uranium utilization, waste management, and proliferation concerns of nuclear energy use.”
In a review of the pathways mapped out to achieve a warming of 1.5⁰C above pre−industrial level by 2050, the IPCC 2018 (Masson−Delmotte et al. 2019, Chapter 2) noted “Nuclear power increases in most 1.5˚C pathways with no or limited overshoot by 2050, but in some pathways both the absolute capacity and share of power from nuclear generators decrease “ (p.131) and “Some 1.5˚C pathways with no or limited overshoot no longer see a role for nuclear fission by the end of the century….” (p. 131). It is noteworthy, however, that in those pathways including nuclear, the contribution of clean renewables is greater than that of nuclear so nuclear is seen, at best, as a minor augmentation to renewable energy.
Porritt (2021) noted that the drive for net zero emissions by 2050 has stimulated the nuclear industry and its proponents to promote that technology as a critical element in achieving the target. His conclusion was summed up simply as: “The problems they face are the same ones that have dogged the industry for decades: ever−higher costs, seemingly inevitable delays, no solutions to the nuclear waste challenge, security and proliferation risks.” This author also makes the important point that “Every kilowatt hour of nuclear−generated power will be a much more expensive kilowatt hour than one delivered from renewables plus storage.” This will compromise low−income Americans more than others.
We seem not to have made much, if any, progress in the nuclear arena over the last 15 years since the Green America (2006) identification of ten reasons to avoid the nuclear option:
- Nuclear waste – waste remains radioactive for tens to hundreds of thousands of years
- Nuclear proliferation − promoting nuclear energy programs increase the likelihood of proliferation of nuclear weapons enhancing the risk of these falling into hands of unstable governments.
- National Security – the danger of nuclear plants serving as targets for aggressor military action and terrorism
- Accidents – cancer rates among populations living in proximity to Chernobyl and Fukushima, especially among children, rose significantly in the years after the accidents,
- Cancer risk – reports of increased cancer risk especially children among populations living near nuclear plants.
- Energy production – in order to provide global energy (nuclear now supplied about 11%), 14,500 plants are required – accompanied by a massive increase in energy−intensive uranium mining.
- Not enough sites – Since nuclear plants require a location near water supplies, there are simply insufficient sites globally to locate 14,500 plants.
- Cost – Unlike cheap renewable energy, nuclear energy is extremely expensive.
- Competition with renewables – Investing in nuclear energy would undermine funding for renewable energy.
- Energy dependence of developing nations – the cost of nuclear would be beyond the capacity of many nations – making them dependent on developed nations for their energy sources. It’s better to promote the far cheaper renewable energy alternative.
Although some evidence suggests that nuclear power may have a valuable role to play, the overall conclusion that the evidence suggests is that nuclear is not necessary, is too dangerous, could not be deployed in the time necessary to solve the crisis, does not lead to GHG emissions reduction, and merely competes for limited investment resources with genuine solutions. The evidence that nations with more versus less nuclear investment produce more greenhouse gas emissions also argues against nuclear energy as a solution to the climate crisis. Given the evidence that we have sufficient Wind, Water, and Solar resources plus storage, to serve our energy demand, we conclude that there is no good reason to divert time and financial resources away from such renewables to bolster a flagging nuclear industry.
SOCAN concurs with Mark Jacobson’s (2021) assessment and his 7 reasons why nuclear energy is not the answer to solve climate change: “New nuclear power costs about 5 times more than onshore wind power per kWh. Nuclear takes 5 to 17 years longer between planning and operation and produces on average 23 times the emissions per unit electricity generated. In addition, it creates risk and costs associated with weapons proliferation, meltdown, mining lung cancer, and waste risks. Clean, renewables avoid all such risks.”
Acknowledgements
For assistance with editing an earlier version of this document, I thank Kathy Conway, Bill Gorham, and Estelle Voeller. I also thank the latter for considerable assistance with ideas and resources on this complex topic. Any errors in the final version, however, are my responsibility.
Literature Cited
Alvarez R, Zavala−Araiza D, Lyon D, Allen D, Barkley Z, Brandt A, Davis K, Scott C. Herndon S, Jacob D, Karion A , Kort E, Lamb B, Lauvaux T, Maasakkers J, Marchese A, Omara M, Pacala S. Peischl J, Robinson A, Shepson P, Sweeney C, Townsend−Small A, Wofsy S, Hamburg S 2018. Assessment of methane emissions from the U.S. oil and gas supply chain. Science 361 (6398) • 186−188. https://www.science.org/doi/10.1126/science.aar7204.
Beyond Nuclear. 2022. Unfounded Promises: Small Modular Reactors (SMRs) solve none of the challenges of nuclear power and make climate change and proliferation worse. Beyond Nuclear Talking Point # 5. https://beyondnuclearinternational.files.wordpress.com/2022/11/bn_talkingpoints6_smallmodularrea ctors_nov2022−1.pdf
Blakemore E 2019 The Chernobyl disaster: What happened, and the long−term impacts. National Geographic, May 17. https://www.nationalgeographic.com/culture/article/chernobyl− disaster#:~:text=International%20researchers%20have%20predicted%20that,may%20suffer%20the%2 0same%20fate.
Bond K, Benham H, Vaughan, Butler E, Sloss S. 2021. The sky’s the limit: Solar and wind energy potential is 100 times as much as global energy demand. Carbon Tracker 71pp. https://carbontracker.org/reports/the−skys−the−limit−solar− wind/?mbid=&utm_source=nl&utm_brand=tny&utm_mailing=TNY_Climate_042821&utm_campaign= aud− dev&utm_medium=email&bxid=5bd673de24c17c104800a1c0&cndid=32390035&hasha=9f3d45e07fc 910bc25840bc92486bca2&hashb=b84e32208904f47e6d9dc356bcb2e7e43d610f98&hashc=b3d65a0a d31dee847d668fe14dac8b9ffbcd841a156dc78401d1c3158e6ec796&esrc=&utm_term=TNY_ClimateCr isis
Bruckner T., Bashmakov I, Mulugetta Y, Chum H, de la Vega Navarro A, Edmonds J, Faaij A, Fungtammasan B, Garg A, Hertwich E, Honnery D, Infield D, Kainuma M, Khennas S, Kim S, Nimir H, Riahi K, Strachan N, Wiser R, and Zhang X. 2014. Energy Systems. (Chapter 7) In: Climate Change 2014: Mitigation of Climate Change. Contribution of Working Group III to the Fifth Assessment Report of the Intergovernmental Panel on Climate Change [Edenhofer O,Pichs−Madruga R, Sokona Y, Farahani E, Kadner S, Seyboth K, Adler A, Baum I, Brunner S, Eickemeier P, Kriemann B, Savolainen J, Schlömer S, von Stechow C, Zwickel T and J.C. Minx (eds.)]. Cambridge University Press, Cambridge, United Kingdom and New York, NY, USA. https://www.ipcc.ch/site/assets/uploads/2018/02/ipcc_wg3_ar5_chapter7.pdf
Brittanica. undated Half−life radioactivity. The Editors of Encyclopaedia Britannica. https://www.britannica.com/science/half−life−radioactivity
Brittanica. 2022. Hydrogen chemical element. Encyclopedia Britannica. https://www.britannica.com/science/technetium
Burkhardt J, Heath G, and Cohen E. 2012. Life Cycle Greenhouse Gas Emissions of Trough and Tower Concentrating Solar Power Electricity Generation. Journal of Industrial Ecology. 16, S93–S109. doi: 10.1111/j.1530. 9290.2012.00474.x, ISSN: 1530−9290.
https://onlinelibrary.wiley.com/doi/full/10.1111/j.1530−9290.2012.00474.x
CDC. 2018. Radioisotope Brief: Iodine−131 (I−131). Radioisotope Emergencies. Centers for Disease Control and Prevention. https://www.cdc.gov/nceh/radiation/emergencies/isotopes/iodine.htm
CDC. 2021. Health Effects of Radiation: Health Effects Depend on the Dose. Radiation and Your Health. Centers for Disease Control and Prevention. https://www.cdc.gov/nceh/radiation/dose.html
Chemeurope. 1997−2022a. Ceasium−135 Chemeurope.com. 1997−2022 LUMITOS AG https://www.chemeurope.com/en/encyclopedia/Caesium−135.html
Chemeurope. 1997−2022b. Isotopes of caesium. 1997−2022 LUMITOS AG https://www.chemeurope.com/en/encyclopedia/Isotopes_of_caesium.html
Chemeurope. 1997−2022c. Palladium 107. Chemeurope.com. 1997−2022 LUMITOS AG. https://www.chemeurope.com/en/encyclopedia/Palladium−107.html
Chemeurope. 1997−2022d, Zirconium 03 Chemeurope.com. 1997−2022 LUMITOS AG. https://www.chemeurope.com/en/encyclopedia/Zirconium−93.html
Clack C, Qvist S, Apt J, Bazilian, M, Brandt A, Caldeira K, Davis S, Diakov V, Handschy M, Hines P, Jafamillo P, Kammen D, Long J, Morgan M, Reed A, Sivaram V, Sweeney J, Tynan G, Victor D, Weyant J, Whitacre J. 2017. Evaluation of a proposal for reliable low−cost grid power with 100% wind, water, and solar. Proceedings of the National Academy of Science. 114 (26) 6722−6727 https://www.pnas.org/doi/10.1073/pnas.1610381114
Clapp R. 2005. Guest Editorial: Nuclear Power and Public Health. Environmental Health Perspectives 113 (11) A720−A721. https://www.ncbi.nlm.nih.gov/pmc/articles/PMC1310934/
Clemmer S, Richardson J, Sattler S, Lochbaum D. 2018. The Nuclear Power Dilemma Declining Profits, Plant Closures, and the Threat of Rising Carbon Emissions Union of Concerned Scientists. https://www.ucsusa.org/sites/default/files/attach/2018/11/Nuclear−Power−Dilemma−full−report.pdf
Cooper, M. 2021. Building a Least−cost, Low carbon Electricity System with Efficiency, Wind, Solar, & Intelligent Grid Management.
https://www.vermontlaw.edu/sites/default/files/2021− 07/Building_a_21st_Century_Electricity_System.pdf
Christodouleas J, Forrest R, Ainsley C, Tochner Z, Hahn S, Glatstein E. 2011. Short−Term and Long−Term Health Risks of Nuclear−Power−Plant Accidents. The New England Journal of Medicine 364:2334−2341. https://www.nejm.org/doi/full/10.1056/nejmra1103676
Depleted UF6. undated. Is Uranium Radioactive? Depleted UF6 Management Information Network. https://web.evs.anl.gov/uranium/faq/uproperties/faq5.cfm#:~:text=The%20half%2Dlife%20of%20ura nium,234%20about%2025%20thousand%20years.
Desbiolles A, Roudier C, Goria S, Stempfelet M, Kairo C, Quintin C, Bidondo M, Monnereau A, Vacquier
B. 2017 Cancer incidence in adults living in the vicinity of nuclear power plants in France, based on data from the French Network of Cancer Registries. https://onlinelibrary.wiley.com/doi/full/10.1002/ijc.31116
DOE 2022. DOE Explains…Deuterium−Tritium Fusion Reactor Fuel. Office of Science, U.S. Department of Energy https://www.energy.gov/science/doe−explainsdeuterium−tritium−fusion−reactor−fuel
Donavan J, Vives P. 2022. Accelerating SMR Deployment: New IAEA Initiative on Regulatory and Industrial Harmonization. International Atomic Energy Agency. https://www.iaea.org/newscenter/news/accelerating−smr−deployment−new−iaea−initiative−on− regulatory−and−industrial−harmonization
Dumé I. 2022. Can thorium compete with uranium as a nuclear fuel? Polytechnique Insights. https://www.polytechnique−insights.com/en/braincamps/energy/the−latest−technological−advances− in−nuclear−energy/can−thorium−compete−with−uranium−as−a−nuclear−fuel/
EERE. Undated a. How Pumped Storage Hydropower Works. Office of Energy Efficiency and Renewable Energy. https://www.energy.gov/eere/water/how−pumped−storage−hydropower−works
EERE Undated b. Pumped Storage Hydropower. Office of Energy Efficiency and Renewable Energy. https://www.energy.gov/eere/water/pumped−storage− hydropower#:~:text=It%20is%20a%20configuration%20of,the%20upper%20reservoir%20(recharge).
EIA. 2021 .Nuclear explained. Nuclear power and the environment. U.S. Energy Information Agency. https://www.eia.gov/energyexplained/nuclear/nuclear−power−and−the− environment.php#:~:text=Nuclear%20energy%20produces%20radioactive%20waste,health%20for%20 thousands%20of%20years
EIA. 2022a. Nuclear Power Plants. Nuclear Explained. U.S. Energy Information Agency. https://www.eia.gov/energyexplained/nuclear/nuclear−power−plants−types−of−reactors.php
EIA. 2022b. Cost and Performance Characteristics of New Generating Technologies, Annual Energy Outlook 2022. U.S. Energy Information Administration. https://www.eia.gov/outlooks/aeo/assumptions/pdf/table_8.2.pdf
EPA. 2021a. Radiation Health Effects. Radiation Protection. United States Environmental Protection Agency https://www.epa.gov/radiation/radiation−health−effects
EPA. 2021b. Radiation Protection − Radionuclide Basics: Technetium−99. United States Environmental Protection Agency. https://www.epa.gov/radiation/radionuclide−basics−technetium−99
EPA. 2022a. About Nuclear Power Plants. Nuclear Power Plants. United States Environmental Protection Agency. https://www.epa.gov/radtown/nuclear−power−plants
EPA. 2022b. Radionuclide Basics: Iodine. Radiation Protection. United States Envionmental Protection Agency. https://www.epa.gov/radiation/radionuclide−basics−iodine
Evans S. 2017 Solar, wind and nuclear have ‘amazingly low’ carbon footprints, study finds. Carbon
Brief. https://www.carbonbrief.org/solar−wind−nuclear−amazingly−low−carbon−footprints
Farmer, M. 2022. Where will the first small modular nuclear reactors be? Power Technology. https://www.power−technology.com/analysis/where−will−the−first−small−modular−nuclear−reactors−be/
Fast Company. 2022. How Far Do You Live From A Nuclear Power Plant? Mansueto Ventures, LLC. https://www.fastcompany.com/3028063/how−far−do−you−live−from−a−nuclear−power−plant
Fernflores F, 2019. The Equivalence of Mass and Energy. Stanford Encyclopedia of Philosophy. https://plato.stanford.edu/entries/equivME/
Fork W, Fowler S. 2021. DOE Requests Comments on the Price−Anderson Act. Pillsbury Insights https://www.pillsburylaw.com/en/news−and−insights/doe−price−anderson−act.html
Fuge L. 2021. What is radioactivity? A source of immense energy with a long and dangerous history. Cosmos Newsletter October 29 2021. https://cosmosmagazine.com/science/physics/what−is− radioactivity/#:~:text=Radiation%20can%20damage%20the%20body’s,sickness%20and%20death%20w ithin%20hours.
Gale P. 2021. Chernobyl: a 35 year follow up on long−term health effects. The Cancer Letter 47 (16) Guest editorial. https://cancerletter.com/guest−editorial/20210423_3/
GE undated Ultra−supercritical and advanced supercritical technology. https://www.ge.com/steam− power/coal−power−plant/usc−ausc
Gray R. 2019. The true toll of the Chernobyl disaster. BBC Future. GE Steam Power. https://www.bbc.com/future/article/20190725−will−we−ever−know−chernobyls−true−death−toll
Green America 2006 10 Reasons to Oppose Nuclear Energy. Green America. https://www.greenamerica.org/fight−dirty−energy/amazon−build−cleaner−cloud/10−reasons−oppose− nuclear−energy
Hatch M, Beyea J, Nieves J, Susser M. 1990. Cancer Near the Three Mile Island Nuclear Plant: Radiation Emissions. American Nournal of Epidemiology, 132 (3) 397−412. https://academic.oup.com/aje/article− abstract/132/3/397/103678?login=false
Holt M. 2018. Price−Anderson Act: Nuclear Power Industry Liability Limits and Compensation to the Public After Radioactive Releases. Congressional Research Service. https://crsreports.congress.gov/product/pdf/IF/IF10821#:~:text=Congress%20responded%20in%2019 57%20by,readily%20available%20within%20those%20limits.
Howarth, R. 2015 Methane emissions and climatic warming risk from hydraulic fracturing and shale gas development: implications for policy. Energy and Emission Control Technologies 2015:3 45–54. https://www.dovepress.com/methane−emissions−and−climatic−warming−risk−from−hydraulic−fracturing−
−peer−reviewed−fulltext−article−EECT
Hpschapters. 2001. Selenium. Argonne National Laboratories. http://hpschapters.org/northcarolina/NSDS/selenium.pdf
Hsu D, O’Donoughue P, Fthenakis V, Heath G, Kim H, Sawyer P, Choi, J and Turney D. 2012. Life Cycle Greenhouse Gas Emissions of Crystalline Silicon Photovoltaic Electricity Generation. Journal of Industrial Ecology Special Issue: Meta−Analysis of Life Cycle Assessments, S122–S135. https://onlinelibrary.wiley.com/doi/full/10.1111/j.1530−9290.2011.00439.x
IAEA. 1998−2022. Radiation in Everyday Life. International Atomic Energy Agency. https://www.iaea.org/Publications/Factsheets/English/radlife#:~:text=The%20ICRP%20recommends% 20that%20any,is%201%20mSv%20per%20year.
IEEFA. 2022. IEEFA U.S.: Small modular reactor “too late, too expensive, too risky and too uncertain.” https://ieefa.org/articles/ieefa−us−small−modular−reactor−too−late−too−expensive−too−risky−and−too− uncertain#:~:text=February%2017%2C%202022%20(IEEFA),Energy%20Economics%20and%20Financial
%20Analysis.
Jacobson M. 2017. 4 Reasons Nuclear and Fossil Fuel Supporters Criticizing 100% Renewable Energy Plan Are Wrong. The Energy Transition − The Global Energiewende. https://energytransition.org/2017/08/4−reasons−nuclear−and−fossil−fuel−supporters−criticizing−100−
renewable−energy−plan−are−wrong/ and Ecowatch https://www.ecowatch.com/pnas−jacobson− renewable−energy−2444465393.html
Jacobson, M. 2019. Evaluation of Nuclear Power as a Proposed Solution to Global Warming, Air Pollution, and Energy Security [In} Jacobson, M. 2020. 100% Clean, Renewable Energy and Storage for Everything. Cambridge University Press, New York, 427 pp., 2020. https://web.stanford.edu/group/efmh/jacobson/Articles/I/NuclearVsWWS.pdf
Jacobson M. 2020 100% Clean, Renewable Energy and Storage for Everything, Cambridge University Press. https://web.stanford.edu/group/efmh/jacobson/WWSBook/WWSBook.html
Jacobson M. 2021. The 7 reasons why nuclear energy is not the answer to solve climate change. Heinrich Bӧll Stiftung, Brussels, European Union. https://eu.boell.org/en/2021/04/26/7−reasons−why− nuclear−energy−not−answer−solve−climate−change
Jacobson M, von Krauland A, Palmer F, Smith M. 2022. Zero air pollution and zero carbon from all energy at low cost and without blackouts in variable weather throughout the U.S. with 100% wind− water−solar and storage. Renewable Energy 184: 430−442. https://linkinghub.elsevier.com/retrieve/pii/S0960148121016499
Jacobson M, Delucchi M, Cameron M, Frew B. 2015. Low−cost solution to the grid reliability problem with 100% penetration of intermittent wind, water, and solar for all purposes. Proceedings of the National Academy of Sciences 112 (49): 15060−15065. https://www.pnas.org/doi/10.1073/pnas.1510028112
Jacobson M, Delucchi M, Cameron M, Frew B. 2017. The United States can keep the grid stable at low cost with 100% clean, renewable energy in all sectors despite inaccurate claims. Proceedings of the National Academy of Sciences. 114 (26) ESO21 – ESO23. https://www.pnas.org/doi/10.1073/pnas.1708069114
Jacoby M. 2020. As nuclear waste piles up, scientists seek the best long−term storage solutions. Chemical and Engineering News 98:12. https://cen.acs.org/environment/pollution/nuclear−waste− pilesscientists−seek−best/98/i12
Kestin G. undated. What does an Atom Look Like. # 18 What the Physics, from Nova. https://www.youtube.com/watch?v=6Wwuvcs3I8g
Kim H, Fthenakis V, Choi J, and Turney D. 2012. Life Cycle Greenhouse Gas Emissions of Thin−film Photovoltaic Electricity Generation. Journal of Industrial Ecology 16, S110–S121. doi: 10.1111/j.1530− 9290.2011.00423.x,ISSN: 1530−9290. https://onlinelibrary.wiley.com/doi/full/10.1111/j.1530− 9290.2011.00423.x
Kinley D III. (Editor) 2003−2005. Chernobyl’s Legacy: Health, Environmental and Socio−Economic Impacts and Recommendations to the Governments of Belarus, the Russian Federation and Ukraine. The Chernobyl Forum: 2003–2005 Second revised version. International Atomic Energy Agency. https://www.iaea.org/sites/default/files/chernobyl.pdf
Koltun P, Tsykalo A, and Novozhilov V. 2018. Life Cycle Assessment of the New Generation GT−MHR Nuclear Power Plant. Energies 2018, 11, 3452; doi:10.3390/en11123452 https://www.mdpi.com/1996− 1073/11/12/3452
Krahn S, Worrall A. 2016. The Reemergence of the Thorium Fuel Cycle: A Special Issue of Nuclear Technology. NUCLEAR TECHNOLOGY · VOLUME 194 · iii–iv · MAY 2016. https://engineering.vanderbilt.edu/cee/GraduateStudy/EnvironmentalEngineering/nuclear−env− engr/JournalArticle2−Krahn−Worrall2016.pdf
Krall l, McFarlane A, Ewing R. 2022. Nuclear waste from small modular reactors. Environmental Sciences May 31, 2022 119 (23) e2111833119 https://www.pnas.org/doi/10.1073/pnas.2111833119
Krzyzaniak j, Brown N. 2019. Fact−check: Five claims about thorium made by Andrew Yang. Bulletin of the Atomic Scientists. December 2019 https://thebulletin.org/2019/12/fact−check−five−claims−about− thorium−made−by−andrew−yang/
Larsen T, 2020. Nuclear Power is Not a Climate Solution. New Labor Forum https://journals.sagepub.com/doi/10.1177/1095796020949231
Lewis M. 2021. NRC Concludes No Major Congressional Changes Necessary to Price−Anderson Act. Up & Atom. https://www.jdsupra.com/legalnews/nrc−concludes−no−major−congressional−9149151/
Libretexts. 2021. 21.7 Nuclear Fission. Libretexts. Chemistry https://chem.libretexts.org/Courses/University_of_Missouri/MU%3A 1330H_(Keller)/21%3A_Nuclea r_Chemistry/21.7%3A_Nuclear_Fission
Liou J. 2021. What Are Small Modular Reactors (SMRs)? International Atomic Energy Authority. https://www.iaea.org/newscenter/news/what−are−small−modular−reactors−smrs
Liou J. 2022. IAEA Initiative Sets Ambitious Goals to Support the Safe and Secure Deployment of SMRs. International Atomic Energy Agency. https://www.iaea.org/newscenter/news/iaea−initiative−sets− ambitious−goals−to−support−the−safe−and−secure−deployment−of−smrs
Lovins A. 2021a. “Low−carbon” misses the point. Beyond Nuclear International.
https://beyondnuclearinternational.org/2021/10/03/low−carbon−misses−the−point/
Lovins A. 2021b. Twelve energy and climate myths. Climate & Capital media. https://www.climateandcapitalmedia.com/twelve−energy−and−climate−myths/
Lyman E. 2019. Aging nuclear plants, industry cost−cutting, and reduced safety oversight: a dangerous mix. Bulletin of the Atomic Scientists, August 2019. https://thebulletin.org/2019/08/aging−nuclear− plants−industry−cost−cutting−and−reduced−safety−oversight−a−dangerous−mix/
Lyman E. 2021. “Advanced” Isn’t Always Better” Assessing the Safety, Security, and Environmental Impacts of Non−Light−Water Nuclear Reactors. Union of Concerned Scientists. https://www.ucsusa.org/sites/default/files/2021−05/ucs−rpt−AR−3.21−web_Mayrev.pdf
Masson−Delmotte, V., Zhai, Pörtner H, Roberts D, Skea J, Shukla P, Pirani A, Moufouma−Okia A, Péan C, Pidcock R, Connors S, Matthews J, Chen Y, Zhou X, Gomis M, Lonnoy E, Maycock T, Tignor M, and Waterfield T. (eds.) 2019. Global Warming of 1.5˚C. An IPCC Special Report on the impacts of global warming of 1.5˚C above pre−industrial levels and related global greenhouse gas emission pathways, in the context of strengthening the global response to the threat of climate change, sustainable development, and efforts to eradicate poverty. https://www.ipcc.ch/site/assets/uploads/sites/2/2019/06/SR15_Full_Report_Low_Res.pdf
Matson J 2011. What Happens During a Nuclear Meltdown? Scientific American, March 2011. https://www.scientificamerican.com/article/nuclear−energy−primer/
Matthews R 2022 Nuclear Power Versus Renewable Energy. The Green Market Oracle. https://thegreenmarketoracle.com/2022/07/20/nuclear−power−versus−renewable− energy/#:~:text=An%20analysis%20of%20the%20levelized,include%20storage%20and%20network%20 costs.
Mirion. 2015. Types on Ionizing Radiation Mirion Technologies. https://www.mirion.com/learning− center/radiation−safety−basics/types−of−ionizing−radiation
Moore S. 2021 Gravity Energy Storage Will Show Its Potential in 2021. January 2021. IEEE Spectrum. https://spectrum.ieee.org/gravity−energy−storage−will−show−its−potential−in−2021
Morelle R. 2022. How close is a fusion−powered future? BBC News https://www.bbc.com/news/science−environment−63950962
Nakhle C. 2022. The potential of small nuclear reactors. Geopolitical Intelligence Services AG https://www.gisreportsonline.com/r/small−modular−reactors/
NAS. 2005. Low Levels of Ionizing Radiation May Cause Harm. National Academy of Sciences. https://www.nationalacademies.org/news/2005/06/low−levels−of−ionizing−radiation−may−cause−harm
NAS. 2006. Health Risks from Exposure to Low Levels of Ionizing Radiation: BEIR VII Phase 2. National Academies of Science. https://nap.nationalacademies.org/catalog/11340/health−risks−from−exposure− to−low−levels−of−ionizing−radiation
NAS. 2022 .Leveraging Advances in Modern Science to Revitalize Low−Dose Radiation Research in the United States. National Academy of Sciences. https://nap.nationalacademies.org/catalog/26434/leveraging−advances−in−modern−science−to− revitalize−low−dose−radiation−research−in−the−united−states
NIH. 2012. Origin of Radioactivity in Nuclear Plants. National Center for Biotechnology Information, National Library of Medicine. https://www.ncbi.nlm.nih.gov/books/NBK201997/
NIRS undated ROUTINE RADIOACTIVE RELEASES FROM NUCLEAR REACTORS − IT DOESN’T TAKE AN
ACCIDENT. Nuclear information Resources Service https://www.nirs.org/wp− content/uploads/factsheets/routineradioactivereleases.pdf
NRC 2021 Fertile Material. United States Nuclear Regulatory Commission. https://www.nrc.gov/reading−rm/basic−ref/glossary/fertile−material.html
NRDC. 2022. Nuclear Power 101 Natural Resources Defense Council https://www.nrdc.org/stories/nuclear−power−101
NREL. 2021. Life Cycle Greenhouse Gas Emissions from Electricity Generation: Update. National Renewable Energy Laboratory. https://www.nrel.gov/docs/fy21osti/80580.pdf
NS Energy. 2018. Major pros and cons of thorium nuclear power reactor. NS Energy https://www.nsenergybusiness.com/news/newsmajor−pros−and−cons−of−thorium−nuclear−power− reactor−6058445/
Nuclear Energy. 2020. NRC Approves First U.S. Small Modular Reactor Design. Office of Nuclear Energy https://www.energy.gov/ne/articles/nrc−approves−first−us−small−modular−reactor−design
Nuclear Matters. 2020. Chapter 15 Nuclear Fuel Cycle and Proliferation. Nuclear Matters Handbook (Revised) https://www.acq.osd.mil/ncbdp/nm/NMHB2020rev/chapters/chapter15.html
Pehl M, Arvesen A, Humpenöder F, Popp A, Hertwich W, Luderer G. 2017. Understanding future emissions from low−carbon power systems by integration of life−cycle assessment and integrated energy modelling. https://www.nature.com/articles/s41560−017−0032−9
Pistilli M. 2022. Are Thorium Reactors the Future of Nuclear Energy? Uranium Investing News https://investingnews.com/daily/resource−investing/energy−investing/uranium−investing/thorium− nuclear−energy/
Polonsky A, Eskelsen G. 2021. NRC Concludes No Major Congressional Changes Necessary to Price− Anderson Act. Up & Atom Blog Post. https://www.morganlewis.com/blogs/upandatom/2021/12/nrc− concludes−no−major−congressional−changes−necessary−to−price−anderson−act
Porritt J. 2021. Don’t believe hydrogen and nuclear hype – they can’t get us to net zero carbon by 2050. The Guardian https://www.theguardian.com/commentisfree/2021/mar/16/hydrogen−nuclear− net−zero−carbon−renewables
Radiation Answers. 2022. Nuclear Power: During normal operations, do commercial nuclear power plants release radioactive material? https://www.radiationanswers.org/radiation−questions− answers/nuclear−power.html
Radioactivity, undated−a. Most fission products vanish quickly. Short−lived fission products. https://www.radioactivity.eu.com/site/pages/Shorl_Lived_Fission_Products.htm
Radioactivity, undated−b. Long−lived Fission Products. A handful of tough but little radioactive isotopes. https://www.radioactivity.eu.com/site/pages/Long_Lived_Fission_Products.htm#:~:text=This%20resid ual%20radioactivity%20of%20fission,and%20technetium%20as%20potential%20troublemakers
Ram M, Bogdanov D, Aghahosseini A, Gulagi A, Oyewo A.S, Child M, Caldera U, Sadovskaia K, Farfan J, Barbosa LSNS, Fasihi M, Khalili S, Dalheimer B, Gruber G, Traber T, De Caluwe F, Fell H, Breyer J.2019 C. Global Energy System based on 100% Renewable Energy – Power, Heat, Transport and Desalination Sectors. 299pp. Study by Lappeenranta University of Technology and Energy Watch Group, Lappeenranta, Berlin. https://ccsi.columbia.edu/sites/default/files/content/docs/EWG_LUT_100RE_All_Sectors_Global_Rep ort_2019.pdf
Richard L. 2013. On Poisoned Ground. Distillations. https://www.sciencehistory.org/distillations/on− poisoned−ground
Rivero N. 2022. America’s green energy hopes hinge on propping up aging nuclear plants. Quartz
https://qz.com/1947873/us−green−energy−efforts−hinge−on−aging−nuclear−plants/
Ross R. 2022. Uranium: Facts about the radioactive element that powers nuclear reactors and bombs. LiveScience. https://www.livescience.com/39773−facts−about−uranium.html
Schellenberger M. 2018. For Nations Seeking Nuclear Energy, The Option To Build A Weapon Remains A Feature Not A Bug. Forbes, August 29th. https://www.forbes.com/sites/michaelshellenberger/2018/08/29/for−nations−seeking−nuclear−energy− the−option−to−build−a−weapon−remains−a−feature−not−a−bug/?sh=2c4403e82747
Science Direct 2022 General Considerations. Cesium 134 Science Direct https://www.sciencedirect.com/topics/medicine−and−dentistry/cesium− 134#:~:text=Cesium%20compounds%20bioconcentrate%20and%20have,Cs%20is%20%E2%88%BC30% 20years.
Sharp T and Bryner J. 2022. Periodic Table of Elements. LiveScience. https://www.livescience.com/25300−periodic−table.html
Skone T, Marriott J, Littlefield J. 2015. Lifecycle Greenhouse Gas Emissions: Natural Gas and Power Production. National Energy Technology Laboratory. https://www.eia.gov/conference/2015/pdf/presentations/skone.pdf
Solutions Project. 2022. Explore our interactive map to see what 100% renewable energy could look like where you live in the year 2050. The Solutions Project https://thesolutionsproject.org/what−we− do/inspiring−action/why−clean−energy/
Soult A. 2020. Fission and Fusion Libretexts. https://chem.libretexts.org/Courses/University_of_Kentucky/UK%3A_CHE_103_−
_Chemistry_for_Allied_Health_(Soult)/Chapters/Chapter_10%3A_Nuclear_and_Chemical_Reactions/1 0.2%3A_Fission_and_Fusion
Sovacool B. 2008. Valuing the greenhouse gas emissions from nuclear power: A critical survey. Energy Policy 36: 2940– 2953. https://www.nirs.org/wp− content/uploads/climate/background/sovacool_nuclear_ghg.pdf
Sovacool B. 2021. Does nuclear power effectively reduce carbon emissions? Beyond Nuclear− Talking Points #3. https://beyondnuclearinternational.files.wordpress.com/2021/05/bn_talking−points_does− nuclear−power−effectively−reduce−carbon−emissions.pdf
Stallard E. 2022. Breakthrough in nuclear fusion energy announced. BBC News https://www.bbc.com/news/science−environment−63950962
Terzic B. 2018.Is power ever too cheap to meter? Atlantic Council: Energy Markets & Governance. https://www.atlanticcouncil.org/blogs/energysource/is−power−ever−too−cheap−to− meter/#:~:text=The%20phrase%20%E2%80%9Ctoo%20cheap%20to,City%20on%20September%2016% 2C%201954.
The Solutions Project. 2022. 100% Renewable Energy Vision https://thesolutionsproject.org/what−we− do/inspiring−action/why−clean−energy/
TMIA. 2019. Study Suggests Three Mile Island Radiation May Have Injured People Living Near Reactor. Three Mile Island Alert. http://www.tmia.com/content/study−suggests−three−mile−island−radiation− may−have−injured−people−living−near−reactor
Torchinsky R. 2022. Solar panels that can generate electricity at night have been developed at Stanford. NPR April 7, 2022. https://www.npr.org/2022/04/07/1091320428/solar−panels−that−can− generate−electricity−at−night−have−been−developed−at−stanf
UF6. Undated. Is uranium radioactive? Depleted YF6. https://web.evs.anl.gov/uranium/faq/uproperties/faq5.cfm#:~:text=The%20half%2Dlife%20of%20ura nium,234%20about%20250%20thousand%20years.
UN News. 2022. Ukraine: Renewed shelling at Zaporizhzhia plant underlines nuclear accident risk. U.N. News Global perspective Human stories. https://news.un.org/en/story/2022/08/1125602
USNRC. 2015. Pressurized Water Reactors. United States Nuclear Regulatory Commission. https://www.nrc.gov/reactors/pwrs.html
Wing S, Richardson D, Armstrong D, Crawford−Brown D. 1997. A reevaluation of cancer incidence near the Three Mile Island nuclear plant: the collision of evidence and assumptions. Environmental Health Perspective 105 (1) 52−57. https://pubmed.ncbi.nlm.nih.gov/9074881/
USNRC. 2021. The Price−Anderson Act: 2021 Report to Congress, Public Liability Insurance and Indemnity Requirements for an Evolving Commercial Nuclear Industry Office (NUREG/CR−7293) United States Nuclear Regulatory Commission https://www.nrc.gov/docs/ML2133/ML21335A064.pdf
USNRC. 2021b. “Too Cheap to Meter”: A History of the Phrase. United Stated Nuclear Regulatory Commission. https://www.nrc.gov/reading−rm/basic−ref/students/history−101/too−cheap−to− meter.html
Veranytsia C, Veranytsia I. 2022 A nuclear risk ‘nightmare’? After seizing Chernobyl, Russian troops exposed themselves to radiation. USA Today, April 20, 2022. https://www.usatoday.com/story/news/world/ukraine/2022/04/20/russia−chernobyl−ukraine−cold− war/7386241001/
Virgili N. 2020. The Impact of Small Modular Reactors on Nuclear Non−Proliferation and IAEA Safeguards. Vienna Center for Disarmament and Non−Proliferation https://www.nonproliferation.eu/wp−content/uploads/2020/08/Virgili−Nicole_SMR−Paper_Final.pdf
Warner E. and Heath G. 2012. Life Cycle Greenhouse Gas Emissions of Nuclear Electricity Generation. Journal of Industrial Ecology 16, S73–S92. doi:10.1111/j.1530−9290.2012.00472.x, ISSN: 1530−9290. https://onlinelibrary.wiley.com/doi/full/10.1111/j.1530− 9290.2012.00472.x#:~:text=LCA%20literature%20indicates%20that%20life,emissions%20relative%20to
Way R, Ives M, Mealy P, Farmer J. 2021 Empirically grounded technology forecasts and the energy transition. Oxford Working Paper No. 2021−01. Institute for New Economic Thinking, The Oxford Martin School. https://www.inet.ox.ac.uk/publications/no−2021−01−empirically−grounded−technology− forecasts−and−the−energy−transition/
Whatisnuclear. 2007−2022a. Thorium as nuclear fuel. Whatisnuclear.com https://whatisnuclear.com/thorium.html
Whatisnuclear. 2007−2022b. Thorium Myths and Misconceptions about Thorium nuclear fuel. Whatisnuclear.com. https://whatisnuclear.com/thorium−myths.html
WNA. 2020. Thorium. World Nuclear Association https://world−nuclear.org/information− library/current−and−future−generation/thorium.aspx
World Nuclear Association. 2021. Plutonium https://world−nuclear.org/information−library/nuclear− fuel−cycle/fuel− recycling/plutonium.aspx#:~:text=Plutonium%20is%20formed%20in%20nuclear,several%20hundred% 20kilograms%20of%20plutonium.
Zielinski S. 2012.What Is Enriched Uranium? Smithsonian Magazine. https://www.smithsonianmag.com/science−nature/what−is−enriched−uranium−17091828/